Next Generation Sequencing vs Sanger Sequencing
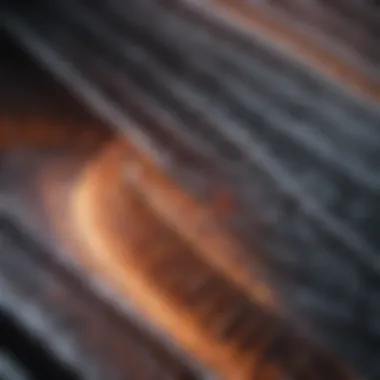
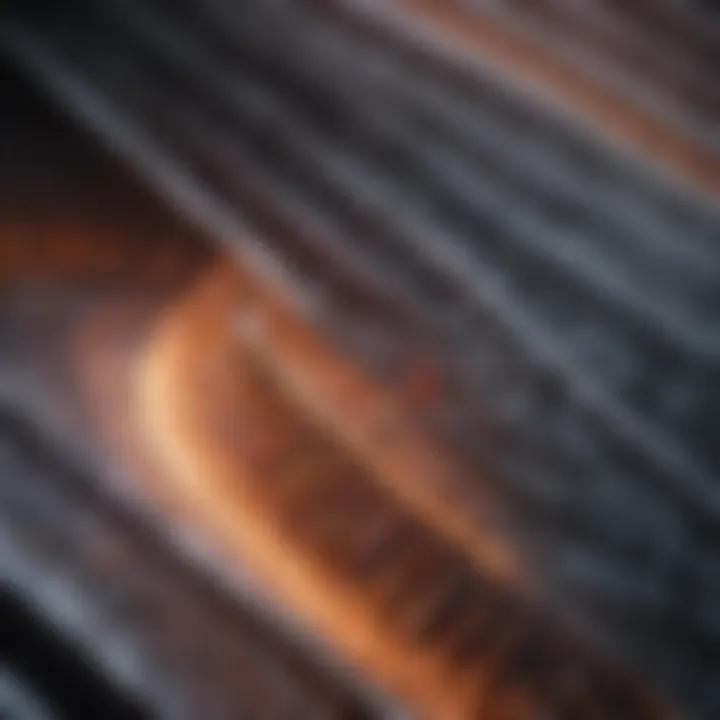
Intro
Next Generation Sequencing (NGS) and Sanger Sequencing are both pivotal technologies in the genomics landscape. Comprehending the differences and similarities between these methods is vital for researchers and students in the field. NGS offers high throughput and rapid data generation, while Sanger sequencing is best known for its accuracy and reliability.
Both techniques have unique methodologies and applications, which can dictate their usage in various genomic studies. Understanding their cost implications, efficiency, and specific applications can guide researchers toward making informed decisions when it comes to selecting a sequencing approach. Analyzing these aspects not only advances individual research projects but also contributes to broader advancements in biological research as a whole.
Preamble to Sequencing Technologies
Understanding sequencing technologies is crucial in the realm of genomics. This section provides an overview of genetic sequencing and emphasizes its significance in the advancement of modern science. These techniques are responsible for unlocking the mysteries of the genome, allowing researchers to explore genetic information in unprecedented detail.
Overview of Genetic Sequencing
Genetic sequencing is the process of determining the precise order of nucleotides within a DNA molecule. DNA sequences are essential for understanding gene function, inheritance patterns, and evolutionary relationships. The methods of sequencing have evolved from the early techniques used in the late 1970s to complex approaches employed today.
There are generally two major methods of sequencing: Sanger sequencing and Next Generation Sequencing (NGS). Sanger sequencing, developed by Frederick Sanger, was the first widely used method. It is characterized by its reliability and accuracy, making it suitable for targeted sequencing projects. In contrast, NGS allows for massively parallel sequencing, offering high throughput, which enables entire genomes to be sequenced in a matter of hours.
Importance of Sequencing in Modern Science
The importance of genetic sequencing in modern science cannot be overstated. This technology has vastly improved our understanding of genetics, leading to breakthroughs in medicine, agriculture, and evolutionary biology. Key areas of impact include:
- Identification of Genetic Diseases: Sequencing aids in identifying genetic mutations that cause hereditary diseases, helping in diagnosis and treatment.
- Personalized Medicine: Through genomic information, treatments can be tailored to the individual, enhancing their effectiveness.
- Research and Development: Sequencing facilitates the study of complex biological processes, aiding research across multiple disciplines.
- Environmental Studies: It helps in tracking genetic variations in populations, providing insight into biodiversity and ecosystem health.
"Sequencing technologies have revolutionized biological research, offering opportunities to explore genetic variation on a scale previously unimaginable."
Sanger Sequencing: Fundamentals
Sanger sequencing is a cornerstone in the field of genetic analysis. It remains vital for understanding DNA sequencing processes. This section investigates the fundamentals underpinning Sanger sequencing, focusing on its historical context and methodological specifics. By examining these areas, the reader can appreciate its role in modern genomics, especially in contrast to newer technologies like Next Generation Sequencing.
Historical Development
Sanger sequencing was developed in 1977 by Frederick Sanger, who pioneered the method to identify nucleotide sequences in DNA. This historical milestone revolutionized genomic research. Before Sanger's innovation, sequencing was a slow and laborious task. Sanger’s approach simplified the process through the use of dideoxynucleotides, which provided a straightforward means of identifying the DNA sequence being studied. This advancement in DNA sequencing was instrumental in the Human Genome Project, providing the necessary technology to decode the entire human genome. Such foundational work underlines the significance of Sanger sequencing in the broader context of genomic advancements.
Methodological Approach
Understanding the methodological approach of Sanger sequencing is crucial for comprehending its strengths and limitations. The method employs a technique known as the chain termination method, which is essential for producing accurate sequence data.
Chain Termination Method
The chain termination method is a pivotal aspect of Sanger sequencing. It functions by incorporating dideoxynucleotides (ddNTPs) during DNA synthesis. These compounds lack the 3'-hydroxyl group necessary for further chain elongation, thus terminating the DNA strand being synthesized. This unique feature allows for a clear delineation of DNA fragments based on size, which can then be separated using gel electrophoresis.
The chain termination method has several merits, including its high accuracy in base calling. However, it does come with drawbacks, such as higher costs and lower throughput compared to Next Generation Sequencing. This balance of accuracy and efficiency is an essential consideration in many genomics studies.
Components Involved
Several components play a significant role in Sanger sequencing, including DNA polymerase, primers, and dideoxynucleotides. The DNA polymerase is pivotal, as it synthesizes the new DNA strand based upon the template provided. Primers are short sequences that provide the starting point for DNA synthesis. Dideoxynucleotides, as mentioned earlier, are crucial for chain termination.
The synergy among these components contributes to the overall functionality of Sanger sequencing. The clarity and precision afforded by this method make it a popular choice for various applications, including clinical diagnostics, validating NGS results, and targeted sequencing. While it may be perceived as less efficient due to throughput constraints, the reliability it offers in specific contexts ensures its continued relevance.
Process Steps
The steps involved in Sanger sequencing are straightforward yet methodically sound. The process can be summarized into several key phases:
- Preparation of DNA Template: DNA is isolated and purified from the sample.
- Primer Annealing: Primers are attached to the template DNA, marking the starting point for duplication.
- Extension and Termination: DNA polymerase synthesizes the new DNA strand, incorporating ddNTPs that cause termination.
- Separation: The resulting fragments of varying lengths are separated using gel electrophoresis.
- Detection and Analysis: Finally, the sequence is analyzed and recorded, often through automated software.
This structured workflow makes Sanger sequencing a formidable technique for precise DNA sequencing, despite its limitations, particularly in terms of scale. The methodology allows for detailed insights into specific gene functions and variations that are particularly beneficial in the study of genetic diseases.
The method's combination of accuracy and reliability makes it paramount in genomics, despite competition from high-throughput technologies.
Next Generation Sequencing: An Overview
Next Generation Sequencing (NGS) represents a significant advance in genomics, offering a powerful alternative to traditional methods such as Sanger Sequencing. This section will lay out key elements, benefits, and considerations surrounding NGS, highlighting its crucial role in modern genomics and biological research.
Preface to NGS
Next Generation Sequencing is a collective term for advanced sequencing technologies that enable the rapid sequencing of large stretches of DNA. Unlike Sanger Sequencing, which provides a single read of a small region of DNA, NGS can process millions of fragments simultaneously. This high-throughput capability drastically reduces the time and costs associated with sequencing, making it attractive for a variety of applications, including whole-genome sequencing and targeted re-sequencing.
The efficiency of NGS is a game changer for researchers, allowing them to analyze complex genomes, identify genetic variants, and explore the multi-faceted nature of biological systems. The scalability and speed of NGS generate vast amounts of data, driving innovative approaches in medicine, agriculture, and evolutionary biology.
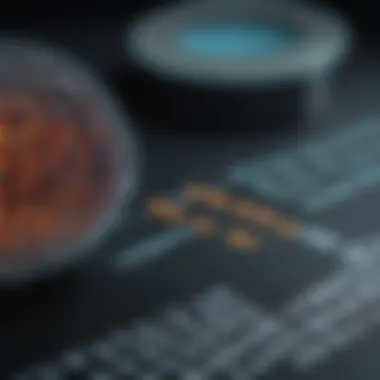
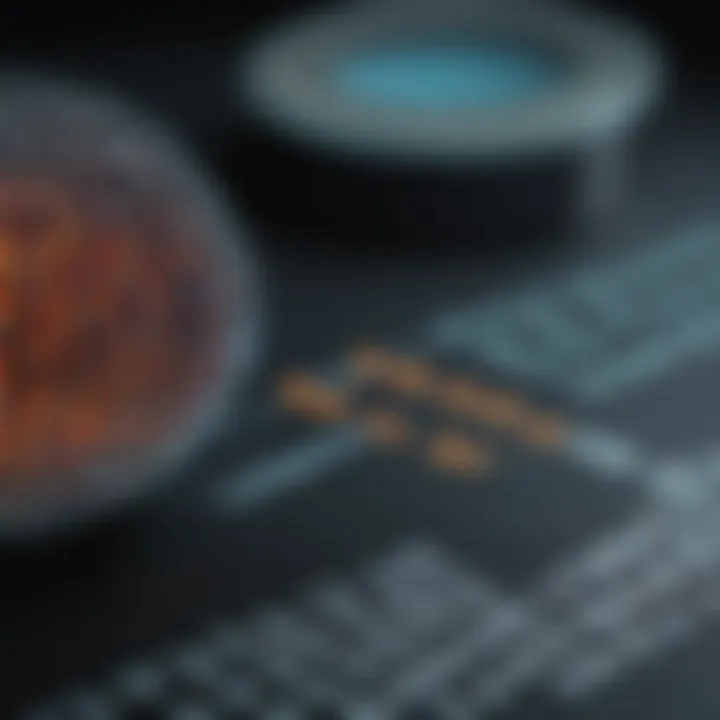
Types of NGS Technologies
Different technologies contribute to the breadth of NGS applications. Here, we will examine three prominent sequencing technologies: Illumina Sequencing, 454 Pyrosequencing, and Ion Torrent Sequencing.
Illumina Sequencing
Illumina Sequencing is the dominant platform in the NGS landscape. Its ability to produce millions of reads in a single run is its most remarkable characteristic. The method uses a reversible dye terminator technology, where fluorescently labeled nucleotides are added, and the sequences are determined from images captured during the sequencing process.
One significant advantage of Illumina Sequencing is its cost-effectiveness. With decreasing costs per base pair, it becomes feasible even for smaller research labs. Additionally, the accuracy rate of over 99% makes it reliable for a variety of applications, including whole-genome, exome, and transcriptome sequencing.
However, Illumina Sequencing is not without limitations. Read lengths are typically short, averaging around 100-300 bases, which can pose challenges during the assembly of complex genomes.
Pyrosequencing
454 Pyrosequencing, once a leading NGS technology, relies on a different chemistr y from Illumina. It uses a sequencing by synthesis approach, wherein the release of pyrophosphate during nucleotide incorporation generates a measurable light signal. This allows for faster real-time sequencing.
The key characteristic of 454 technology is its longer read lengths, often up to 600 bases, which offers advantages in sequencing through repetitive regions that can confuse shorter reads. This makes it a favorable option for certain applications, such as de novo sequencing of complex genomes and metagenomic studies.
Despite its strengths, 454 Pyrosequencing has seen a decline in popularity due to higher costs and lower throughput compared to Illumina.
Ion Torrent Sequencing
Ion Torrent Sequencing represents another innovative approach by detecting the release of hydrogen ions during DNA polymerization. This capacitor-based technology is distinct from optical methods, making it faster and less expensive to operate.
A significant advantage of Ion Torrent Sequencing is the rapid turnaround time, often completing sequencing runs in just a few hours. This real-time capability appeals to clinicians and researchers needing quick results.
On the downside, the accuracy can be affected by homopolymer regions, where the sequencing may struggle to determine the exact number of repeated nucleotides. Thus, careful consideration of its application is necessary in contexts where sequencing precision is crucial.
In summary, each NGS technology brings unique strengths and challenges that must be weighed against specific research goals. Understanding these can enhance informed decision-making in genomics research.
Comparative Analysis of Both Techniques
The comparative analysis of Sanger Sequencing and Next Generation Sequencing (NGS) forms the backbone of understanding their distinctive features. In this article, we delve into the nuances of each technique, examining specific elements that drive their use in genomics. The relevance of this comparison is pronounced, since informed decisions in sequencing methods can significantly impact research outcomes and efficiency.
Throughput and Scalability
Throughput, or the amount of data produced in a given timeframe, distinguishes sequencing technologies fundamentally. Sanger Sequencing is known for its relatively low throughput. While it's effective for smaller projects, the constrained capacity can limit its application in larger studies. Typical Sanger sequencing can produce approximately 1,000 to 5,000 base pairs per run. This may suffice for targeted applications, such as single-gene analysis, but fails for extensive genomic studies.
In contrast, NGS immensely improves throughput and scalability, allowing for millions of DNA fragments to be sequenced simultaneously. Technologies like Illumina and Ion Torrent can sequence whole genomes at unprecedented speeds and efficiencies, generating gigabases of data in a single run. This capability transforms research possibilities, allowing for large-scale projects such as population genomics and cancer studies.
However, the increased throughput of NGS comes with the need for robust data management systems. The generated data requires careful handling to ensure accurate analysis and interpretation.
Accuracy and Error Rates
Accuracy is critical in sequencing, directly influencing downstream applications. Sanger Sequencing boasts high accuracy rates, commonly exceeding 99%. This remarkable precision makes it a reliable choice for clinical diagnostics and validating results from NGS. Its error profile, mostly involving substitutions, is well understood, allowing for effective error-correction strategies.
NGS, however, presents a more complex accuracy landscape. While it offers high throughput, the error rates can be more variable, often between 0.1% to 1%. Issues include substitution errors, insertions, and deletions, which can complicate the interpretation of results. Various techniques are in place to mitigate these errors, such as multiple sequencing runs and algorithms designed for error correction. Understanding these differences is vital for researchers as they determine which method to employ based on their project's requirements.
Read Length Considerations
Read length is a significant factor in sequencing technology, affecting how well complex genomes can be assembled or analyzed. Sanger Sequencing traditionally provides longer reads, generally around 800 to 1,000 base pairs, which facilitates accurate alignments and reduces the ambiguity in repetitive regions of the genome.
Conversely, NGS typically offers shorter reads, ranging from 50 to 600 base pairs, depending on the specific technology used. While these shorter reads enable high-throughput sequencing, they can present challenges in genomic assembly and analysis. Overlapping reads are essential for reconstructing complex genomic regions, which can be complicated by the shorter length. In situations requiring high-quality assemblies, longer reads, such as those from third-generation sequencing technologies, might be advantageous. Researchers must assess the implications of read length based on their specific projects and goals, weighing the benefits of NGS's high-throughput capabilities against the needs for accurate assemblies.
Applications of Sanger Sequencing
Sanger Sequencing plays a vital role in various biological and medical applications. Despite the emergence of Next Generation Sequencing technologies, Sanger Sequencing remains a gold standard due to its reliability and accuracy. This section discusses key applications where Sanger Sequencing is particularly important, highlighting its unique benefits and considerations.
Clinical Diagnostics
Sanger Sequencing is widely used in clinical diagnostics to identify genetic mutations linked to diseases. Its high accuracy makes it suitable for characterizing specific gene variants. In conditions like cystic fibrosis or certain cancers, pinpointing mutations can inform treatment decisions and genetic counseling.
- Detection of Single Nucleotide Variants (SNVs): Sanger Sequencing is effective for identifying SNVs. These changes can have significant impacts on protein function and ultimately on patient health.
- Targeted Mutation Analysis: Many diagnostic tests focus on a known set of mutations related to certain genetic diseases. Sanger's ability to analyze specific regions of DNA makes it ideal in these scenarios.
- Confirmation of Results: After initial screening, Sanger Sequencing is often used to confirm the presence of mutations detected by other methods, such as Next Generation Sequencing. This step is crucial in clinical settings where accurate diagnosis is essential.
Sanger Sequencing's established protocols and regulatory approvals facilitate its integration into clinical labs. Therefore, it offers reliable results when detecting mutations in genetic disorders.
Validation of NGS Results
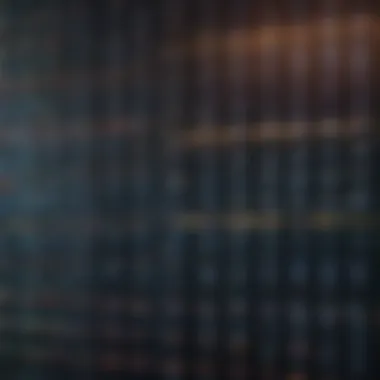
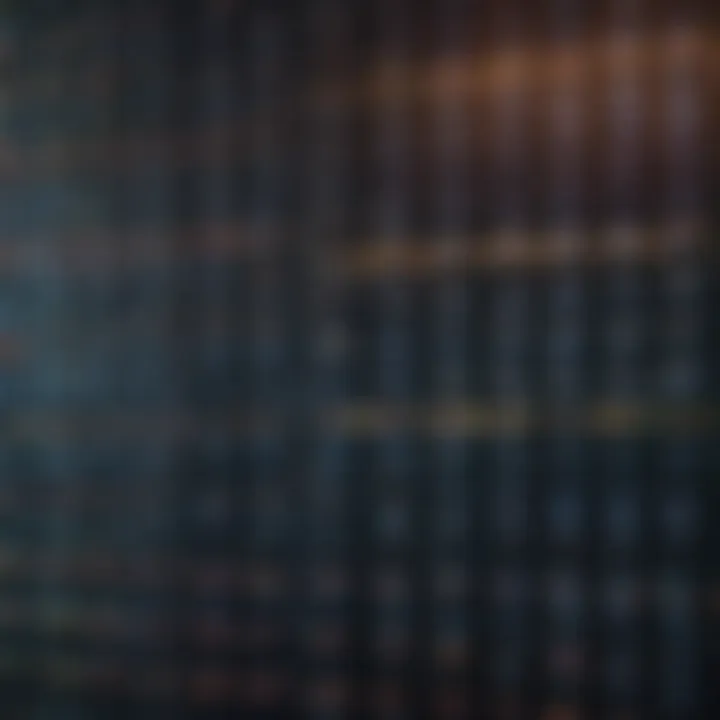
With the growing implementation of Next Generation Sequencing means, there can be uncertainties about results due to its complex nature. Sanger Sequencing acts as a crucial tool for validating outcomes from NGS.
- Cross-Verification: Results obtained from NGS can be ambiguous, especially in variant interpretation. Sanger Sequencing is often employed to cross-verify the presence or absence of specific mutations detected by NGS.
- Error Resolution: NGS might have higher error rates with low-frequency variants. Sanger Sequencing’s precision ensures that clinically significant mutations are accurately confirmed.
- Reassuring Outcomes in Research: In research contexts, Sanger Sequencing provides a dependable method to confirm findings from larger population studies or clinical trials harnessed through NGS.
By integrating Sanger Sequencing for validation, researchers and clinicians enhance the credibility of their findings and ensure patient safety.
"Sanger Sequencing's role in validating NGS results reinforces its relevance in modern genomics, even as newer technologies emerge."
In summary, the applications of Sanger Sequencing in clinical diagnostics and validation of NGS results underline its importance in contemporary genetics. It provides specificity and accuracy that are vital to genomic analysis, proving essential in advancing personalized medicine.
Applications of Next Generation Sequencing
Next Generation Sequencing (NGS) has revolutionized various fields in genomics and has become an essential tool in research and clinical settings. Its applications are diverse, ranging from understanding complex diseases to advancing personalized medicine. This section outlines the specific applications of NGS, emphasizing its benefits and the considerations researchers must take into account.
Whole Genome Sequencing
Whole Genome Sequencing (WGS) entails the comprehensive sequencing of an organism's entire genome. This technique provides critical insights into genomic variations, including single nucleotide polymorphisms (SNPs) and structural variants. Therefore, WGS is invaluable in a wide range of applications:
- Disease Research: Researchers utilize WGS to identify genetic mutations linked to diseases. This contributes significantly to discovering rare genetic disorders.
- Population Genomics: WGS aids in assessing genetic diversity across populations, allowing for better understanding of evolutionary biology.
- Personalized Medicine: By analyzing an individual’s genome, clinicians can tailor treatment plans based on the genetic predisposition of patients. This approach enhances treatment efficacy and minimizes adverse effects.
The comprehensive nature of WGS means it generates significant data. Therefore, data management systems must adapt to handle large datasets efficiently.
Targeted Sequencing
Targeted Sequencing focuses on specific areas of the genome, rather than sequencing the entire genome. This method is advantageous when researchers aim to study particular genes or regions known to be associated with specific diseases.
The benefits of this approach include:
- Cost-Effectiveness: Targeted panels can be sequenced at a fraction of the cost of WGS. This makes it more accessible for many laboratories and clinicians.
- Time Efficiency: The targeted nature of this sequencing allows for quicker results, facilitating timely clinical decisions.
- Higher Coverage: By focusing on specific genes, targeted sequencing can achieve higher coverage, enhancing the detection of variants.
Despite these advantages, incorrect target selection can hinder valuable insights. Thus, thorough pre-sequencing analysis is crucial.
Metagenomics Studies
Metagenomics entails sequencing genetic material directly from environmental samples, allowing researchers to explore microbial communities without the need for culturing. This application has wide-reaching implications in various fields:
- Microbiome Research: Metagenomics helps characterize diverse microbial populations within human bodies, which can influence health and disease outcomes.
- Environmental Monitoring: Researchers can assess biodiversity and the effects of environmental changes by studying microbial communities in ecosystems.
- Biotechnology: Metagenomics provides insights useful for drug discovery and development, leading to new therapeutics derived from microorganisms.
However, metagenomics generates complex datasets, requiring robust analytical tools for interpretation. The integration of bioinformatics with metagenomics is pivotal for meaningful conclusions.
In summary, the applications of NGS are vast and continue to expand. Each application has its advantages and considerations, underscoring the importance of NGS in impactful genomic research.
Cost Considerations
Cost considerations are a significant aspect of any genomic study. It helps researchers and institutions make informed decisions about sequencing methods based on their specific needs and budgets. Understanding the financial implications of using Sanger Sequencing versus Next Generation Sequencing is crucial in determining the suitable approach for various applications in genomics. The cost can influence which method is chosen, thereby impacting research outcomes and their overall feasibility.
Cost of Sanger Sequencing
Sanger Sequencing is often considered the gold standard of sequencing methods, especially for smaller-scale projects. The cost of implementing Sanger Sequencing is generally higher per base pair sequenced when compared to its counterpart, Next Generation Sequencing. This cost typically includes:
- Reagents and Consumables: The materials for the chain-termination method are more expensive on a per-sample basis.
- Laboratory Time: Skilled personnel are usually required to execute the process, substantially adding to the overall expenditure.
- Equipment Maintenance: The need for well-maintained equipment also plays a critical role in budgeting for Sanger Sequencing.
In laboratory settings, the costs may vary, but estimates can be approximately $500 to $2000 per sample depending on the target size and complexity.
Cost of Next Generation Sequencing
Next Generation Sequencing has revolutionized the cost model for genomic studies. The pricing structure is more favorable for larger projects due to its high throughput capabilities. Some factors impacting the cost of NGS includes:
- Platform Choice: Different NGS platforms, such as Illumina or Ion Torrent, can have varied pricing structures, affecting the overall investment required.
- Batch Processing: NGS allows for multiple samples to be sequenced concurrently, which significantly reduces the cost per sample.
- Data Storage: The larger datasets produced by NGS necessitate adequate data storage solutions, which can represent a hidden cost.
Overall, NGS has brought costs down to around $1000 to $2000 for a whole human genome, making it more economically viable for large-scale studies.
Cost-Effectiveness Comparison
When comparing cost-effectiveness between Sanger and NGS, it is evident that they serve different purposes and target distinct research areas:
- For Low-Throughput Projects: Sanger Sequencing may still be justified for smaller studies due to its precision and reliable accuracy.
- For Large Studies or Whole Genomes: NGS is the preferred method because of its ability to process large volumes of data at a lower cost per base.
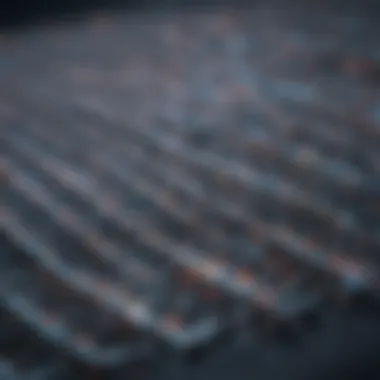
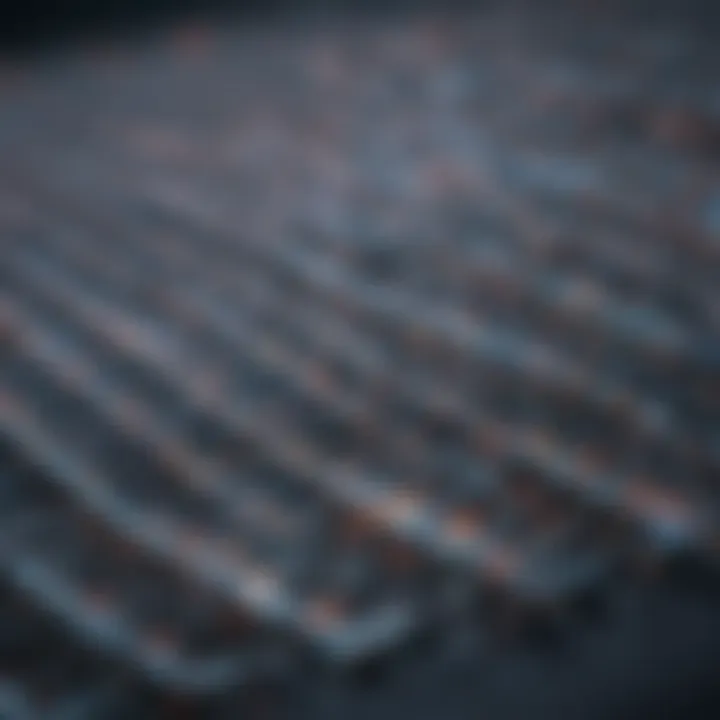
In summary, while Sanger Sequencing has its merits, particularly in clinical applications, Next Generation Sequencing's scalability and cost-effectiveness make it favorable for many exploratory and comprehensive genomic studies.
"The choice between Sanger and Next Generation Sequencing is often dictated by the specific research requirements, expected data output, and budget constraints. Each technique offers unique advantages and limitations that need careful consideration."
Limitations of Sanger Sequencing
While Sanger sequencing has been a crucial method in genomics, it does possess several limitations that researchers must consider. Understanding these limitations is essential for making informed decisions about sequencing methodologies. This section will discuss the throughput limitations and time consumption, two fundamental challenges associated with Sanger sequencing.
Throughput Limitations
Sanger sequencing can handle relatively small volumes of samples at once. Typically, it can generate data for several hundred bases of a single DNA fragment per reaction. This is substantially lower than what Next Generation Sequencing (NGS) technologies can achieve. For instance, NGS can sequence millions of fragments simultaneously, enabling it to cover entire genomes in a fraction of the time. Consequently, Sanger sequencing becomes impractical for large-scale projects such as whole genome sequencing or large population studies.
Additionally, the process requires individual reactions for different DNA segments, creating a bottleneck in throughput. As the demand for high-volume data increases in research, the limits of Sanger sequencing's throughput become even more pronounced. Researchers looking to accelerate discovery should be aware that relying solely on Sanger sequencing may hinder their ability to process large datasets efficiently.
Time Consumption
The time required for Sanger sequencing is another critical limitation. The method involves multiple steps, including DNA amplification, purification, and sequencing reactions. Each of these phases can be time-consuming, often taking from several hours to days. This protracted timeline contrasts sharply with NGS techniques, which can produce results in just a few days or even hours, depending on the scale of sequencing.
Moreover, because Sanger sequencing is slower and requires manual intervention at various stages, it is less suitable for applications needing rapid results, such as clinical diagnostics or real-time surveillance of pathogens. Researchers and laboratories need to balance the accuracy of Sanger sequencing with its time demands, especially when rapid decision-making is critical.
In summary, while Sanger sequencing has its merits, limitations in throughput and time consumption may drive researchers to seek alternative methods like Next Generation Sequencing for more extensive and timely data analysis.
Limitations of Next Generation Sequencing
Next Generation Sequencing (NGS) has revolutionized the approach to genomics. However, it is essential to acknowledge the limitations inherent within this methodology. Addressing these limitations provides insight into the precise conditions where NGS may be less favorable compared to other techniques, particularly Sanger Sequencing. Understanding these constraints helps researchers and practitioners navigate the complexities of genomic studies effectively.
Technical Complexity
One significant limitation of NGS lies in its technical complexity. The setup for NGS can be intricate and demanding, requiring specialized equipment and expertise. These requirements can be a barrier for smaller laboratories or institutions that might not have access to advanced technologies. The workflow also includes several stages, such as library preparation, sequencing, and data analysis, each with its own specific protocols and potential points of failure.
The library preparation process, for instance, is critical for the quality of the NGS results. It involves fragmenting DNA and attaching adapters, a procedure susceptible to contamination and errors. Moreover, different NGS platforms have unique requirements and specifications. This adds an additional challenge, as protocols may need customization to fit specific sequencing technologies.
Data Management and Analysis
The second primary limitation relates to data management and analysis. NGS produces vast amounts of data, often referred to as "big data" in genomics. This data is not just large in volume but also complicated in structure. Processing and storing this information demands substantial computational resources and sophisticated data analysis tools. Consequently, this aspect may overwhelm laboratories lacking computational facilities.
Effective interpretation of NGS data also necessitates advanced bioinformatics skills. Researchers need to utilize various algorithms and software capable of parsing the data accurately. Issues may arise with data quality, alignment, and variant calling, requiring critical knowledge to distinguish between true biological variations and artifacts introduced during the sequencing process.
"The explosion of data generated by NGS represents both a remarkable opportunity and a formidable challenge for the scientific community."
Future Perspectives
Understanding the future perspectives in sequencing technologies is essential for researchers and practitioners in the field of genomics. This section outlines upcoming trends and developments that may influence the landscape of genetic sequencing. As the demand for precise and efficient sequencing grows, researchers must stay informed about these advancements. They allow for improved methodologies and broader applications, addressing not only the scientific community but also public health and genomic medicine.
Advances in Sequencing Technologies
Recent years have witnessed exciting advancements in sequencing technologies, moving the field closer to high-accuracy, high-throughput solutions. Techniques such as single-molecule real-time sequencing (SMRT) and nanopore sequencing demonstrate the potential of direct sequencing without amplification. These advances promise more rapid and accurate results, with the ability to generate long reads that can enhance assembly for complex genomes. Furthermore, the continuing miniaturization of sequencers makes them more accessible in various laboratory settings.
Integration of Technologies
The integration of sequencing technologies with computational biology offers significant potential to enhance genomic research. Combining data from both Next Generation Sequencing and Sanger Sequencing could lead to more robust findings. For example, using Sanger methods for validation of NGS results can improve confidence in variant calling. This hybrid approach allows researchers to leverage the strengths of both techniques, thereby achieving a more comprehensive insight into genomic data.
Expanding Applications and Impacts
The applications of sequencing technologies are expanding across numerous fields, from personalized medicine to environmental microbiology. With advances in sequencing, researchers can conduct more extensive studies, such as population genomics and evolutionary biology. The implications are significant, as precise genomic data can guide public health strategies and conservation efforts. The future of sequencing also suggests potential advancements in early cancer detection, rare disease diagnosis, and even infectious disease control through better understanding of pathogen genomes.
"The advancements in sequencing are reshaping our understanding of biology and medicine, paving the way for more effective treatments and preventive measures."
Overall, future perspectives on sequencing technologies highlight an exciting potential for innovation. Continuous research and development are necessary to expand their integration and applications, creating transformative opportunities in science.
End
The conclusion of an article on Next Generation Sequencing and Sanger Sequencing serves as an essential recap of the discussions throughout the text. It allows the reader to consolidate their understanding of both methodologies and appreciate their relative strengths and weaknesses. This section emphasizes the importance of choosing the right sequencing technology based on specific needs, the intended application, and the research objectives.
Key Takeaways
- Distinct Methodologies: Sanger sequencing is a well-established technique, characterized by its chain termination method. Meanwhile, Next Generation Sequencing offers higher throughput and scalability, essential for large-scale projects.
- Cost-Effectiveness: When evaluating these technologies, cost considerations are crucial. NGS often offers a more economical solution for large sample sizes, while Sanger sequencing remains valuable for smaller, precise applications.
- Applications: Each method has unique applications. Sanger sequencing is preferred in clinical settings for specific gene analysis, while NGS has revolutionized fields such as whole genome sequencing and metagenomics.
- Future Directions: Technological advancements in both areas will likely enhance their relevance in various fields, ensuring they continue to meet the demands of modern scientific inquiries.
Making Informed Choices in Sequencing
Selecting the appropriate sequencing technology necessitates a clear understanding of specific research requirements. Researchers must consider factors such as:
- Objective of the Study: Is the goal to sequence a small set of genes or an entire genome? This decision will greatly influence the choice between Sanger and NGS.
- Budget Constraints: Financial resources available for the project can restrict options. Understanding the overall costs associated with each method is crucial.
- Data Complexity: The ability to process and analyze the generated data efficiently is another factor. NGS produces massive amounts of data, requiring robust bioinformatics capability.
- Accuracy Needs: For projects where precision is paramount, Sanger sequencing is often preferred due to its higher fidelity.
In summary, comprehensively reflecting on these elements will lead to better informed decisions when it comes to choosing sequencing methodologies. The nuances and implications of each technology must be weighed carefully, ensuring the selected option aligns with the specific goals of the research.