Exploring Gene Expression and Transcription Mechanisms
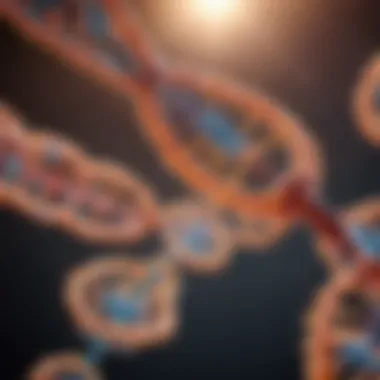
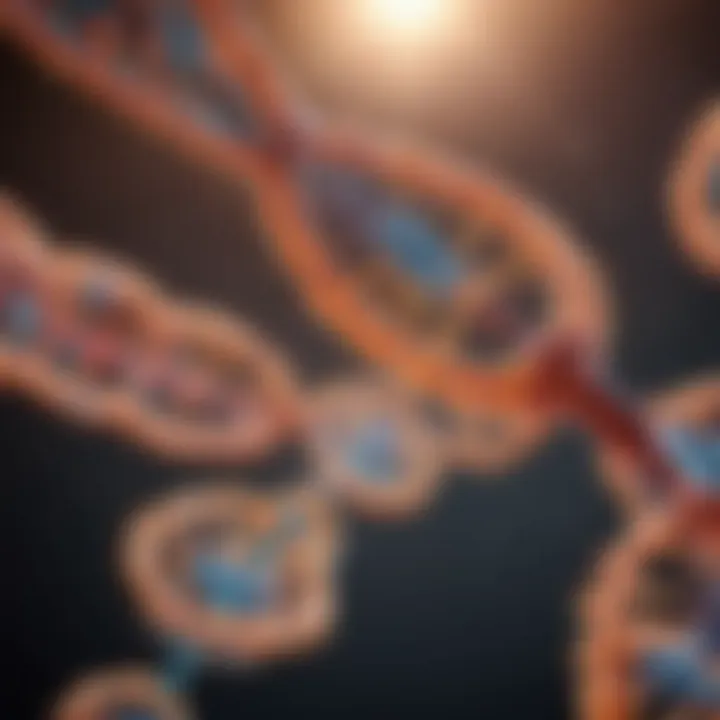
Intro
Gene expression is not just a biochemical curiosity; it influences everything from developmental pathways to responses to environmental cues. This exploration invites us to consider how tiny variations in gene expression can lead to wide-ranging effects, ultimately shaping an organism's health and disposition. As we embark on this journey through the stages of transcription and the various factors influencing these intricate mechanisms, let’s keep in mind their implications in day-to-day biological functions and diseases.
In this section, we will navigate through core concepts, unraveling the complexities of the transcription process that serve as the pathway to gene expression. By diving deep into the stages and regulators involved, we aim to paint a clear picture of how life’s instructions are interpreted and executed at the molecular level.
Intro to Gene Expression
Gene expression is foundational in biology, underpinning the very nature of life itself. When we speak about gene expression, we are referring not just to the biological process—it's about understanding how genes turn into functional products like proteins, which are the workhorses of the cell. It's a dynamic dance, where signals from inside and outside the cell influence the timing and degree of gene activity.
Grasping the concept of gene expression is crucial for a myriad of reasons. It’s the linchpin for understanding developmental biology, cellular responses to environmental changes, and even the etiology of diseases. For instance, the expression profiles of certain genes can dictate whether a cell remains a stem cell or differentiates into a specialized cell type. Thus, researchers digging into gene expression phenomena can potentially unlock therapies for various diseases, making it a hotbed for investigative endeavors.
Definition of Gene Expression
In simplest terms, gene expression is the process through which the genetic code—from DNA—is converted into the functional products that carry out specific tasks in the body. Typically, this involves two main steps: transcription and translation. During transcription, DNA is used as a template to synthesize messenger RNA (mRNA). This mRNA then serves as the blueprint that gets translated into proteins. Understanding this sequence is more than academic; it's about recognizing how our bodies function at a molecular level, impacting everything from metabolism to immune response.
"Gene expression can be viewed as the orchestra of life, where DNA is the sheet music, RNA is the conductor, and proteins are the musicians playing their parts."
Historical Context
The story of gene expression evolves alongside our understanding of genetics itself. At the turn of the 20th century, Gregor Mendel laid the groundwork with his pea plant experiments, revealing patterns of inheritance that hinted at a deeper molecular basis. Fast forward to the mid-20th century, the discovery of the double helix by Watson and Crick and the subsequent elucidation of the genetic code marked significant milestones. Researchers began to see the stitch that links genetic information to biological processes.
In the ensuing decades, advancements in molecular biology brought forth techniques that allowed scientists to isolate, study, and manipulate genes. The Central Dogma of molecular biology, articulated by Francis Crick, simplified the understanding of genetic flow from DNA to RNA to protein, further grounding the significance of gene expression.
As the understanding of proteins and their functions expanded, it became clear that gene expression is not static. It can be influenced by factors like stress, nutrition, and exposure to toxins. In recent years, the rise of technologies—including CRISPR gene editing and RNA sequencing—has opened new avenues for research, paving the way for even deeper insights into the intricacies of gene expression.
In summary, delving into gene expression is not just an academic exercise; it's a journey through the essence of biology, significantly contributing to our grasp of health and disease, echoing its relevance across many fronts of science and medicine.
The Biological Importance of Gene Expression
Gene expression serves as the very backbone of biological systems, acting as a finely-tuned orchestra where each note plays a crucial role in the symphony of life. This section highlights the significance of gene expression, showcasing its essential functions and far-reaching implications in various biological contexts. From cellular functions to developmental processes, understanding gene expression is paramount for deciphering the intricate workings of life itself.
Role in Cellular Function
At the core of cellular functionality is gene expression, dictating how cells interpret and respond to their environments. Imagine a bustling city where each worker follows specific instructions to maintain order and functionality; similarly, in a cell, genes provide the instructions needed to produce proteins that serve various roles. These proteins can be enzymes, signaling molecules, or structural components necessary for maintaining cellular integrity.
When a gene is expressed, it typically results in the transcription of mRNA, which is then translated into a protein. This protein can carry out diverse functions, such as facilitating metabolic processes, aiding in cellular repair, and regulating the cell cycle. The precise timing and level of gene expression determine how effectively a cell functions.
Consider a muscle cell, which requires the elevation of specific proteins for contraction and movement. The regulation of gene expression allows muscle cells to respond swiftly to signals for growth or repair, adapting to varying demands. Likewise, in immune cells, the expression levels of genes enable a rapid response to infections.
"Gene expression is the genetic blueprint that drives both the day-to-day activity and long-term survival of all living organisms."
Impact on Development and Differentiation
Gene expression plays a pivotal role during the intricate processes of development and differentiation. From a single fertilized egg, a highly organized multicellular organism emerges, with distinct cell types performing specialized functions. This transformation is orchestrated by the precise activation and repression of genes in specific patterns.
In developmental biology, the concept of cell differentiation is critical. For example, stem cells are like blank canvases, capable of becoming any cell type depending on which genes are turned on or off at various stages. The differentiation of these cells dictates the formation of tissues and organs, each tailored to perform specific functions necessary for survival.
The signaling pathways and gene regulatory networks involved in determining cell fate are complex; they ensure that cells receive the right signals at the right times. Through misregulation of gene expression, however, developmental anomalies may arise. These could manifest as congenital disorders, where certain tissues or organs do not develop correctly.
In summary, the biological importance of gene expression cannot be overstated. It serves as the foundation for cellular function and the driving force behind development and differentiation, laying the groundwork for both normal physiology and the intricacies of disease.
Overview of Transcription
Transcription is a critical step in the process of gene expression, acting as a bridge between the information contained in DNA and the synthesis of proteins that dictate cellular functions. Understanding transcription is fundamental for grasping how genes are turned on or off, allowing cells to respond to environmental signals and maintain homeostasis. This overview delineates the mechanics of transcription, highlighting its stages, implications in various biological pathways, and importance in research and clinical settings.
In essence, transcription is the first step in decoding genetic information. By converting DNA into messenger RNA (mRNA), it sets the stage for subsequent translation into proteins. If we liken the DNA to a cookbook containing recipes for various dishes, transcription serves as the act of copying a single recipe onto a notepad—making it accessible and ready for cooking.
Defining Transcription
Transcription can be defined simply as the process by which the information in a segment of DNA is copied into a complementary RNA molecule. This occurs in the nucleus for eukaryotic cells, while in prokaryotes it occurs in the cytoplasm. At its core, transcription serves to generate the blueprints required for the synthesis of proteins, which carry out a vast array of functions within an organism.
Stages of Transcription
Transcription can be divided into three crucial stages: initiation, elongation, and termination. Each stage plays a pivotal role in ensuring that the genetic information is expressed accurately and effectively.
Initiation
Initiation is the first step in transcription, where the process begins with the binding of RNA polymerase to the promoter region of a gene. This stage is designated by its critical nature of setting transcription in motion. The key characteristic of initiation is the recognition of specific DNA sequences by transcription factors which ultimately recruit RNA polymerase. This makes initiation a fundamental point of control in gene expression, as it determines whether transcription will take place.
A unique feature of initiation involves the pre-initiation complex formation, entailing various proteins and cofactors. This complexity adds robustness to the transcription process, ensuring that genes are expressed in response to specific developmental or environmental cues.
Elongation
Once initiation has occurred, elongation takes center stage. In this phase, RNA polymerase moves along the DNA template strand, synthesizing mRNA by adding complementary RNA nucleotides. The unmatched pace of elongation, often several thousand nucleotides per minute, is a key characteristic, enabling the rapid production of mRNA necessary for cellular functions.
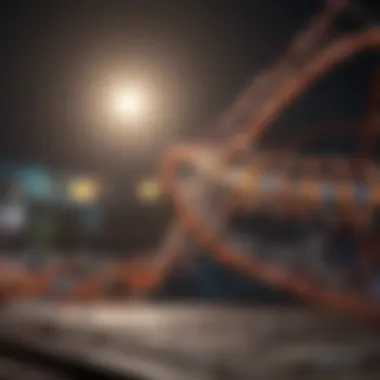
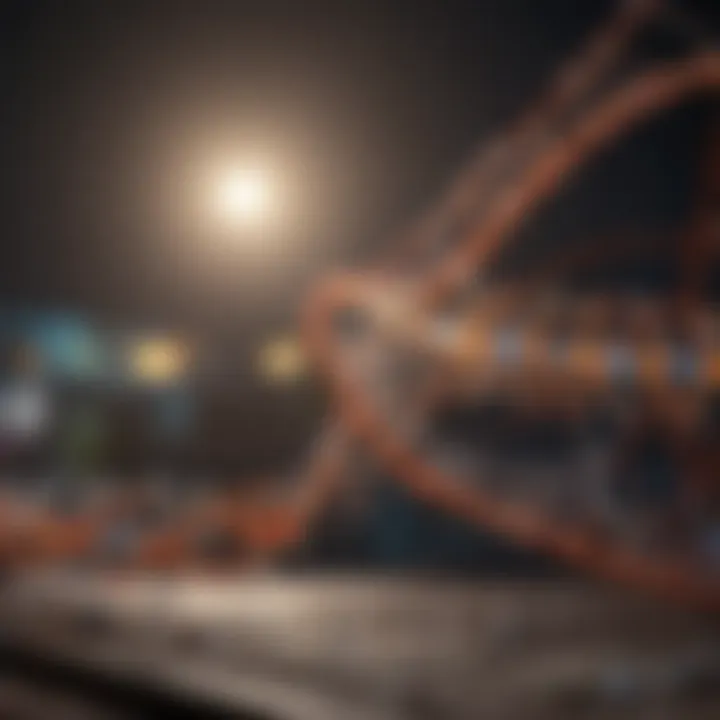
A unique feature of elongation is the formation of a transcription bubble, where the DNA strands separate and allow RNA synthesis to proceed. This phase enhances the efficiency of mRNA production but also carries risks—incorrect nucleotide incorporation could lead to mutations.
Termination
Termination marks the conclusion of the transcription process. This stage occurs when RNA polymerase reaches specific sequences in the DNA, signaling it to detach from the DNA strand and release the newly synthesized mRNA molecule. The significance of termination is underscored by its role in ensuring that transcripts are produced in a precise and regulated manner.
An interesting aspect of termination is the mechanism involved; there are two primary types: intrinsic and rho-dependent termination. Each method has its own unique features and implications for gene expression efficiency.
"In an organism, the efficiency of transcription is paramount, guiding how cells respond and adapt to ever-changing scenarios inherent to life itself."
Understanding each of these transcription stages is essential for appreciating how genes are expressed and regulated. In the greater scheme, transcription is not just a mere copy-and-paste job; it's a finely tuned orchestra that relies heavily on its players—transcription factors, RNA polymerases, and even the DNA being transcribed. Each part must perform flawlessly to result in accurate gene expression.
Molecular Players in Transcription
In the grand orchestration of gene expression, transcription stands as a pivotal act hinged on various molecular players. Comprehending who these players are and how they function is essential. They not only ensure that genetic instructions are effectively communicated from DNA to RNA but also shape the very essence of cellular behavior. This section will dive deep into these integral components: the DNA template, RNA polymerase enzymes, and nucleotides, elucidating their unique roles and the symphony they create in transcription.
DNA as the Template
At the heart of transcription is DNA, serving as the essential template. This double-helix marvel houses the genetic instructions necessary for life. It is one of those things that seem so simple, yet the breadth of its complexity is staggering. During transcription, a specific segment of DNA unwinds and separates, making those precious genes accessible.
Each gene has its own unique sequence, which directly facilitates the synthesis of mRNA.
- Antisense Strands: In this process, the antisense strand of DNA is used, which complements the sequence of the mRNA being formed.
- Promoter Regions: These regions signal where transcription should begin, like a green light on a busy intersection.
This meticulous opening-up process not only ensures that the right information is transcribed but also positions the cell for intricate regulatory controls that affect gene expression levels.
RNA Polymerase Enzymes
Next up in this transcription drama are the RNA polymerase enzymes. Picture them as the diligent scribes, faithfully transcribing the information encoded within DNA. There are several types of RNA polymerase, each with its own specialization, but for transcription, we predominantly discuss RNA polymerase II in eukaryotic cells, which is responsible for synthesizing mRNA.
These enzymes carry a few remarkable features:
- Catalytic Activity: RNA polymerases catalyze the addition of ribonucleotide triphosphates to the growing RNA strand, elongating it with precision.
- Processivity: They can synthesize lengthy RNA sequences without detaching from the DNA template, which is crucial for accurate transcription.
- Complex Interaction: They rely on a myriad of additional proteins to initiate transcription properly, demonstrating that teamwork makes the dream work.
As they traverse the DNA template, RNA polymerases also monitor the integrity and quality of the growing RNA strand, ensuring fidelity in the genetic message being conveyed.
Role of Nucleotides
Then we have the nucleotides, the building blocks of RNA. These petite molecules might seem too small to matter, but don't let that fool you. They're the lifeblood of transcription. Just like bricks in a wall, they come together to construct RNA strands, essential for carrying genetic information.
- Types of Nucleotides: Each nucleotide comprises a nitrogenous base (adenine, uracil, cytosine, or guanine), a ribose sugar, and a phosphate group. The nitrogenous base is what sets each nucleotide apart, determining the sequence of the transcribed RNA.
- Triphosphate Form: Nucleotides in transcription are typically in triphosphate form, providing the necessary energy for the incorporation into the growing RNA chain.
- Base Pairing: They also dictate the specificity of the RNA message, pairing with complementary DNA bases in the template strand.
Thus, while they are small, their impact is significant. Without nucleotides, the transcription process wouldn’t even get off the ground.
"The harmony of these molecular players is crucial, shaping not only the transcript but the very fate of cell function itself."
Regulatory Elements in Gene Expression
Regulatory elements in gene expression play a pivotal role in the intricate dance of biological processes. Without these elements, the precise regulation that creates the diverse array of cellular functions we observe would be a chaotic affair. We could think of them as the backstage crew of a theater production—essential for the performance, yet often unnoticed by the audience.
These elements comprise a variety of sequences in the genome that dictate how and when genes are expressed. Their presence or absence can significantly affect the outcome of gene expression, thus influencing everything from cell behavior to overall organism development.
The benefits of understanding these regulatory elements cannot be understated. They hold keys to insights into various biological phenomena, especially in health and disease. Recognizing how these elements operate allows scientists to develop targeted therapies, assist in genetic engineering, and advance our understanding of evolutionary biology.
But with this importance comes a host of considerations. For example, how these elements interact with one another, the specific signaling pathways involved, and the environmental factors influencing their activity are all crucial aspects that require comprehensive exploration.
Promoters and Enhancers
Promoters and enhancers are fundamental regulatory sequences that work like skilled conductors leading an orchestra. They control the initiation and amplification of transcription. Promoters are typically located directly upstream of a gene and contain specific sequences that RNA polymerase and transcription factors recognize and bind to, thus starting the transcription process. These sequences essentially act as a landing strip for the machinery required for gene expression.
On the other hand, enhancers are more like distant cheerleaders that can be located far away from the genes they regulate. They boost the transcription levels by helping transcription factors interact with the promoter, facilitating contact over vast stretches of DNA. This interaction can happen through looping mechanisms that draw distant regions of the DNA together, highlighting the dynamic nature of the genome.
Silencers and Insulators
In contrast, silencers and insulators serve as the brakes and barriers in this regulatory landscape. Silencers actively repress gene expression, effectively telling RNA polymerase to take a detour. These elements can be found near the promoters or even further away. When recognized by specific proteins, silencers can hinder access to the transcription machinery, ensuring that certain genes remain silent when they should be.
Insulators, meanwhile, play a crucial role in maintaining the integrity of gene expression patterns. They act like fences that prevent enhancers from activating neighboring genes, thus maintaining a clear boundary. By doing so, they organize the genome into distinct functional domains, allowing cells to establish unique identities based on the specific expression profiles appropriate for their functions.
The interplay between promoters, enhancers, silencers, and insulators can dictate cellular fates in a remarkably precise manner, underlining the complexity of gene regulation.
Overall, regulatory elements are integral to understanding gene expression. As we peel back the layers of cellular and molecular mechanisms, the complex interplay of these elements reveals much about how life remains regulated and robust, adapting to various challenges and changes in the environment. As research continues to expand, these insights will pave the way for innovative therapeutic strategies and enhanced understanding of biological processes.
Transcription Factors and Their Roles
Transcription factors are essential proteins that bind to specific DNA sequences, playing a pivotal role in the regulation of gene expression. They can act as "on" switches, enhancing gene expression, or as "off" switches, curtailing it. This dual functionality is crucial for maintaining the delicate balance of cellular processes and responding to changes in the environment.
Activators and repressors are types of transcription factors that have opposing functions. Activators enhance the transcription of specific genes, effectively increasing protein production. Their mechanisms often involve interactions with enhancer regions of the DNA, where they facilitate the assembly of the transcription machinery. In contrast, repressors can hinder transcription by binding to silencer regions or directly interacting with the general transcription factors to inhibit gene expression.
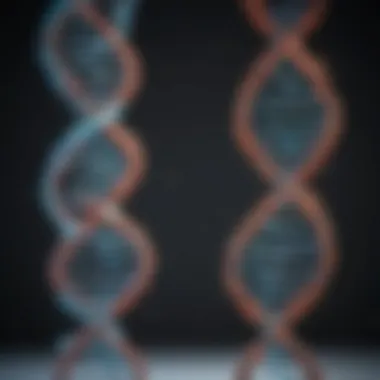
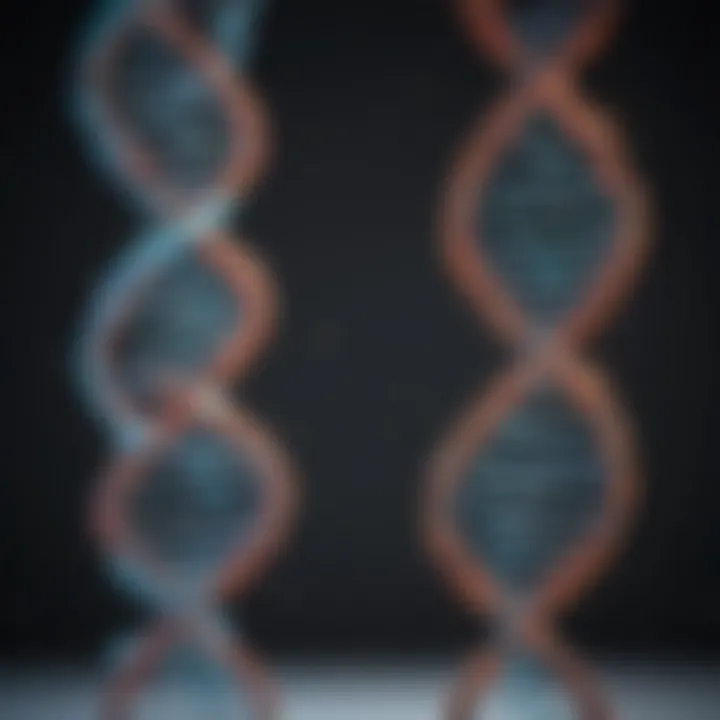
Understanding how these transcription factors operate is essential for grasping the complexities of gene regulation. They don't function in isolation; rather, they integrate signals from various pathways to fine-tune gene activity.
"Transcription factors are the gatekeepers of gene expression, choosing which genes get the spotlight in the cell's performance."
Activators and Repressors
Activators are typically characterized by their ability to stimulate the transcription of specific target genes. These factors can recruit additional proteins, such as coactivators, that help to remodel chromatin and make DNA more accessible to the transcriptional machinery.
On the flip side, repressors play a crucial role in keeping potentially harmful gene expression in check, especially in contexts where gene products could lead to improper function or pathways. Repressors may also influence chromatin structure to lock down access to the DNA, thereby halting unwanted transcription. Some key points about these two types of transcription factors include:
- Modulation of Cellular Responses: Activators typically respond to signaling molecules, adjusting the transcription of genes based on environmental needs, while repressors can prevent detrimental effects by silencing genes at inappropriate times.
- Complex Interactions: Both activators and repressors often interact with other proteins, forming complexes that either promote or inhibit gene expression.
- Diverse Roles Across Systems: These transcription factors are not limited to specific genes or pathways - they play versatile roles across various cell types and organisms.
Interactions with Other Proteins
Transcription factors do not act alone. They share a stage with other proteins to facilitate an elaborate network of gene regulation. This interaction is essential for ensuring that transcription adheres to the precise timing and location necessary for proper cellular function.
One prominent aspect of transcription factor interaction is the cooperation with coactivators and repressors. Coactivators assist in assembling the transcriptional machinery, often enabling the activators to make contact with the basal transcription apparatus. Some well-studied coactivators include the Mediator complex, which acts as a bridge between transcription factors and RNA polymerase II.
Conversely, repressors can form complexes with other proteins, which may act to impede the function of activators. This antagonistic relationship creates a balanced regulatory environment, vital for numerous physiological processes.
In summary, understanding the roles of transcription factors, activators, and repressors offers valuable insights into the sophisticated mechanisms behind gene expression. Their interconnected relationships, involving intricate protein interactions and regulatory feedback loops, further underscore the complexity of cellular regulation. This knowledge is not only foundational for genetics but also has significant implications in the fields of molecular biology and medicine.
Post-Transcriptional Modifications
Post-transcriptional modifications are crucial steps that occur after the transcription of DNA into messenger RNA (mRNA). These alterations are more than just a finishing touch; they significantly influence how genes are expressed, affecting everything from RNA stability to translation efficiency. Essentially, these modifications help tailor the mRNA for its eventual role in protein synthesis, ensuring that cells function optimally and respond effectively to their environment. As gene expression can be modified at many stages, understanding post-transcriptional modifications offers important insights into cellular processes.
Capping
One of the first modifications enacted post transcription is capping. This process involves the addition of a modified guanine nucleotide at the 5' end of the newly synthesized mRNA. Capping has several key functions:
- Protection from degradation: The cap structure shields mRNA from enzymatic degradation by exonucleases, which otherwise could undermine mRNA stability.
- Facilitation of translation: The cap is essential for the binding of ribosomes during translation. Without it, the mRNA may not be recognized by the ribosomal machinery.
- Regulation of nuclear export: Capping is also a signal to facilitate the export of mRNA from the nucleus to the cytoplasm.
In summary, capping is not a mere decoration—it is a critical modification that significantly affects mRNA longevity and functionality.
Polyadenylation
Following capping, polyadenylation occurs at the 3' end of the mRNA, involving the addition of a tail made up of adenine nucleotides, commonly referred to as the poly(A) tail. This process is not only essential but of high importance itself due to several reasons:
- Increased stability: Similar to capping, the poly(A) tail protects the mRNA from degradation, allowing it to persist longer in the cytoplasm and thus more available for translation.
- Influence on translation efficiency: The length of the poly(A) tail can also determine how efficiently the ribosome can translate the mRNA into a protein. Longer tails tend to enhance translation rates.
- Nuclear export assistance: The addition of this tail helps to signal the mRNA for export out of the nucleus.
Ultimately, polyadenylation serves as a critical checkpoint, shaping the overall fate of mRNA in the cellular context.
Splicing
Splicing is perhaps the most intricate of post-transcriptional modifications. It involves the removal of non-coding sequences called introns from the mRNA transcript while retaining coding sequences known as exons. This process not only generates a mature mRNA molecule ready for translation, but it also has several implications:
- Diversity of protein products: Alternative splicing allows for multiple protein isoforms to be produced from a single gene, increasing the diversity of proteins that can be generated and allowing cells to fine-tune their functional responses.
- Regulatory mechanisms: The splicing process is regulated by a variety of factors, including splicing factors and regulatory proteins, which can impact how exons are joined together. This regulation plays a critical role in gene expression control.
- Influence on mRNA stability: The manner in which splicing is conducted can affect mRNA stability and thus its availability for translation.
Splicing is like a meticulous editing process, ensuring the final mRNA product is not just functional but also optimized for its specific cellular duties.
The integration of these post-transcriptional modifications highlights the sophistication of gene expression regulation. Each step is vital to ensure that the genetic material is expressed precisely when and where it is needed.
Techniques in Studying Gene Expression
Exploring the techniques in studying gene expression is vital to understanding how genes are regulated and how they function within cells. Precise measurement of gene expression helps scientists unravel the mysteries of cellular processes. Dipping into these techniques not only reveals the pathways and mechanisms involved but also underscores the impact on medical science, agriculture, and biotechnology. With every leap in technology, a clearer view of gene interaction emerges, propelling research towards practical applications in various fields.
Quantitative PCR
Quantitative PCR, or qPCR, is a go-to method for measuring the abundance of specific RNA transcripts. It's like having a magnifying glass on the genetic material, allowing researchers to see which genes are turned on or off in given conditions. This technique offers several priceless benefits:
- High Sensitivity: It can detect minute quantities of nucleic acids, ensuring that even small fluctuations in expression levels are captured.
- Specificity: With the right primers, researchers can focus on specific genes, avoiding the noise that comes from unwanted targets.
- Quantitative Nature: Unlike traditional PCR, qPCR provides quantitative data, offering clear insights into gene expression levels.
However, it’s important to consider the limitations. Sample quality, primer design, and overall experimental design can influence results significantly. Small changes may seem monumental without proper controls, making validation critical.
Microarrays
Microarrays represent another landmark technique in studying gene expression. They provide a platform that can measure the expression levels of thousands of genes simultaneously, making it an efficient option for large-scale genomic studies. At its core, the microarray technique involves:
- Hybridization: Fluorescently labeled cDNA or RNA samples hybridize to complementary sequences fixed on a chip.
- Data Analysis: Detection systems then measure the intensity of fluorescence, translating it into expression levels for each gene.
These capabilities allow for comparative analyses in varied conditions, such as healthy vs. diseased tissues, giving crucial insights into pathologies. However, microarrays can sometimes yield false positives due to cross-hybridization, which necessitates careful validation of results. Furthermore, the static nature of microarrays limits their ability to capture dynamic changes in gene expression over time.
RNA Sequencing
RNA sequencing, commonly referred to as RNA-seq, is a technique that has revolutionized the ways we study gene expression. Unlike its predecessors, RNA-seq provides a comprehensive picture by sequencing entire mRNA populations. Here are some standout features of RNA-seq:
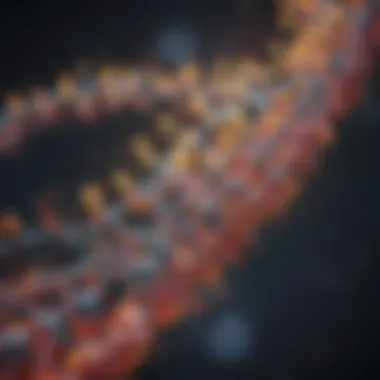
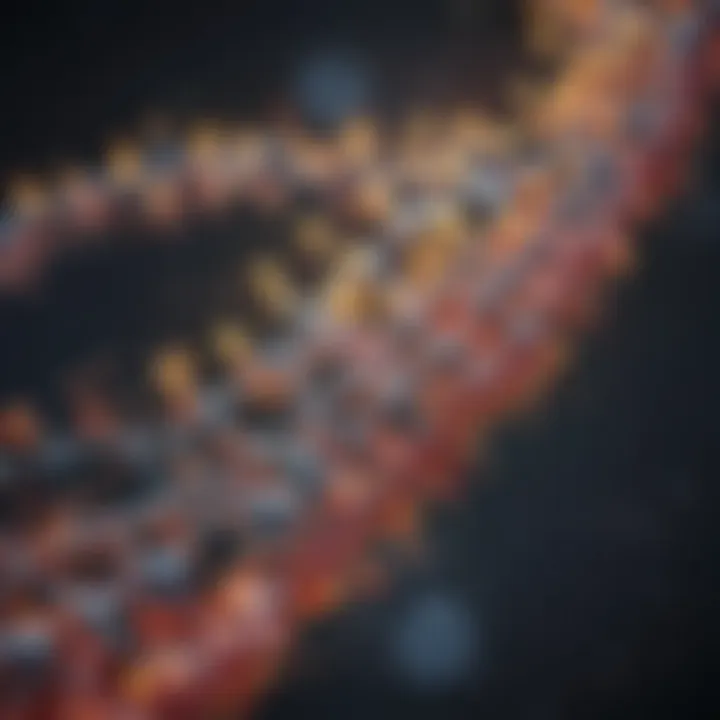
- Unbiased Detection: It captures all transcripts, providing insights into novel transcripts, splice variants, and even non-coding RNA.
- Dynamic Range: The method's broad dynamic range accommodates both lowly expressed and highly expressed genes, offering a more accurate reflection of cellular states.
- Single-Cell Resolution: Recent advancements allow researchers to look at gene expression in individual cells, unveiling the heterogeneity within cellular populations.
Nevertheless, the complexity of data generated can be a double-edged sword. It requires sophisticated computational tools and expertise for analysis. With many processing and filtering steps, it's easy to introduce biases if one isn’t vigilant.
"The techniques we choose to study gene expression can shape our understanding of health and disease, both in the lab and in clinical settings."
In summary, each technique brings forth its unique strengths and challenges. From the precision of quantitative PCR to the vast possibilities offered by RNA sequencing, understanding gene expression hinges on effectively employing these methods. As technologies evolve, so too will our insight into the intricate world of genes and their expressions.
Clinical Implications of Transcription Regulation
Gene transcription is not just a molecular ballet taking place inside the confines of the cell; it has real-life implications that ripple throughout the fabric of health and disease. Understanding the nuances of transcription regulation helps unravel the complexities surrounding various medical conditions and can pave the way for innovative treatments.
The ability of cells to control gene expression through transcription influences a host of biological processes, crucially affecting cellular behavior. This regulation comes into play in several clinical contexts, making it an essential area of study.
Oncogenes and Tumor Suppressors
The delicate balance of gene expression is often disrupted in cancer, and this disruption typically involves the interplay of oncogenes and tumor suppressor genes. Oncogenes are mutated forms of normal genes (proto-oncogenes) that, when activated, drive cancerous growth. Essentially, these genes hasten the cell cycle, pushing cells to divide unchecked. A classic example is the HER2 gene, which, when overexpressed, is associated with aggressive breast cancers.
On the flip side, tumor suppressors work to restrain cell division and promote normal cellular function. An archetypal tumor suppressor gene is TP53, famously dubbed the "guardian of the genome" due to its role in preventing the survival of cells with damaged DNA. Mutations in such genes can lead to a loss of control over the cell cycle, contributing to tumor formation.
- Key Points to Consider:
- The overexpression of oncogenes can lead to aggressive treatment-resistant cancers.
- Loss of function in tumor suppressors means that critical checkpoints in the cell cycle are ignored, which can urge normal cells to transform into cancerous ones.
- Identifying these genes offers potential targeted therapies. For example, drugs targeting the BRAF mutation help in certain melanoma cases.
Cancer is like a puzzle, built by mistakes in transcription regulation. Figure out the pieces, and you might just find a solution.
Genetic Disorders and Mutations
The ramifications of transcriptional regulation extend beyond cancer; they can also provide insights into various genetic disorders. Mutations in regulatory regions, transcription factors, or the genes themselves can lead to developmental disorders or syndromes.
For instance, mutations affecting the regulation of FOXP2, a gene crucial for speech and language, can result in profound language impairments, aptly named developmental verbal dyspraxia. Similarly, alterations in transcription factors such as SOX10 can play a role in conditions like Waardenburg syndrome, which can lead to hearing loss and pigmentation changes.
- What to Keep in Mind:
- Genetic disorders often emerge from subtle shifts in the regulatory network that governs gene expression.
- Understanding these mechanisms aids in the identification of biomarkers for early detection and diagnosis.
- Advances in gene therapy are already showing promise; for example, CRISPR technology offers innovative ways to correct transcriptional errors at their source.
Presumably, the deeper one dives into the molecular underpinnings of diseases, the more they realize that transcription regulation is akin to the conductor of an orchestra, ensuring that each section plays its part in harmony. Unraveling these complexities is not only fascinating from a scientific standpoint but pivotal for meaningful clinical outcomes.
Emerging Research in Gene Expression
Emerging research in gene expression is at a pivotal point, bringing fresh perspectives and cutting-edge techniques to the forefront of molecular biology. This segment addresses the exciting developments currently shaping our understanding of gene expression, focusing particularly on CRISPR, epigenetics, and single-cell transcriptomics. Each of these fields is not only expanding our comprehension of gene regulation but is also providing innovative avenues for therapeutic interventions.
CRISPR and Gene Editing
CRISPR, or Clustered Regularly Interspaced Short Palindromic Repeats, along with its associated proteins like Cas9, has revolutionized the field of gene editing. Its ability to target specific sequences of DNA with remarkable precision has garnered substantial attention in both research and clinical settings. Through gene editing, scientists can now modify genetic material, turning genes on or off or even correcting mutations that cause diseases. This technology is considered a game-changer for developing treatments for genetic disorders such as cystic fibrosis or sickle cell anemia.
The implications of CRISPR in gene expression studies are immense. Researchers are now able to dissect the functions of individual genes by silencing or activating them, allowing a clearer understanding of their roles in cellular processes. Moreover, CRISPR also opens doors for exploring complex regulatory networks and interactions that were previously difficult to probe. The rapid advancement of CRISPR technology continues to stir ethical discussions about its application in humans, sparking debates on the morality of gene editing.
Epigenetics and Transcription
Another frontier in gene expression research is epigenetics—the study of changes in gene activity without altering the DNA sequence. Epigenetic modifications, such as DNA methylation and histone modification, play critical roles in regulating gene expression by influencing the chromatin structure and accessibility of transcription machinery to DNA. This means that environmental factors such as diet, stress, and toxins can leave a mark on our DNA that may affect gene expression and overall health.
Investigating epigenetics is crucial not only for understanding fundamental biological processes but also for its potential to inform public health strategies. For instance, lifestyle interventions aimed at altering epigenetic markers could offer preventive measures against diseases like obesity and diabetes. The link between epigenetics and transcription is becoming clearer as studies show how these modifications can enhance or silence the transcription of genes, highlighting a complex interplay that dictates cell function.
Single-Cell Transcriptomics
Finally, single-cell transcriptomics represents a significant leap in research methodologies, allowing for the analysis of gene expression at a single-cell resolution. Unlike traditional bulk RNA sequencing, which only provides an average expression profile across a population of cells, single-cell transcriptomics reveals the diversity of gene expression patterns within heterogeneous cell mixtures. This technology is particularly beneficial for understanding complex tissues such as the brain or tumors, where specific cell types may have pronounced differences in gene expression.
By using single-cell RNA sequencing (scRNA-seq), researchers can identify rare cell populations, investigate developmental trajectories, and understand how cells respond to environmental changes at an unprecedented level of detail. The insights gained can lead to better diagnostic tools and targeted therapies, potentially improving clinical outcomes significantly.
"The future of genomics lies not just in the information contained within our DNA, but also in the layers of regulation that govern how genes are expressed," says Dr. Smith, a leading expert in molecular biology.
In summary, the exploration of emerging research in gene expression is an ongoing journey. The interplay of technologies like CRISPR, the insights offered by epigenetics, and breakthroughs in single-cell transcriptomics shine a light on the intricate mechanisms that govern gene regulation and expression, laying the groundwork for future innovations in medicine and biotechnology.
End
In the grand tapestry of molecular biology, the conclusion serves as a pivotal moment to reflect on the journey through gene expression and transcription processes. Ultimately, understanding these mechanisms isn't just an academic pursuit; it has tangible implications on health, disease, and biotechnology.
Summary of Key Points
Throughout this article, we have covered numerous aspects of gene expression and transcription. Key takeaways include the following:
- Gene expression shapes cellular identity and function by regulating which proteins are made and in what quantities.
- Transcription, the initial step in gene expression, is a complex process involving multiple stages: initiation, elongation, and termination.
- Various molecular players, including RNA polymerase and transcription factors, play essential roles in overseeing and fine-tuning the transcription process.
- Post-transcriptional modifications like capping, polyadenylation, and splicing are crucial for the stability and functionality of RNA molecules.
- Recent advances in research methodologies such as CRISPR and single-cell transcriptomics are paving the way for new discoveries in gene expression mechanics and therapeutic applications.
Future Directions in Research
The journey doesn’t end here. The future of research in gene expression and transcription shows promise in several exciting avenues:
- Investigating the role of epigenetics: Understanding how environmental factors influence gene expression through epigenetic modifications adds a layer of complexity to our understanding.
- Exploring transcriptional dynamics: Using cutting-edge techniques to measure how quickly and efficiently genes are expressed can provide deeper insights into cell behavior.
- Implications of non-coding RNAs: Research into non-coding RNA molecules, which play regulatory roles, could lead to breakthroughs in understanding gene silencing and modulation.
- Therapeutic applications: As we improve our understanding of the regulatory elements that control gene expression, the potential for gene therapies and precision medicine becomes increasingly viable.
"Gene expression is the heartbeat of cellular life; understanding it opens the door to countless innovations in health and biology."
As we blend molecular insights with clinical applications, the implications for understanding gene expression continue to expand, offering a wealth of opportunities for future explorations. The study of transcription isn’t merely a look at how genes are activated; it’s a window into the very essence of life itself.