Understanding Protein Synthesis: Transcription & Translation
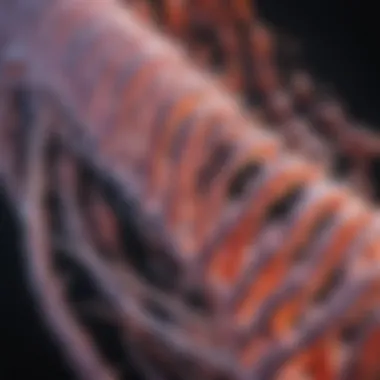
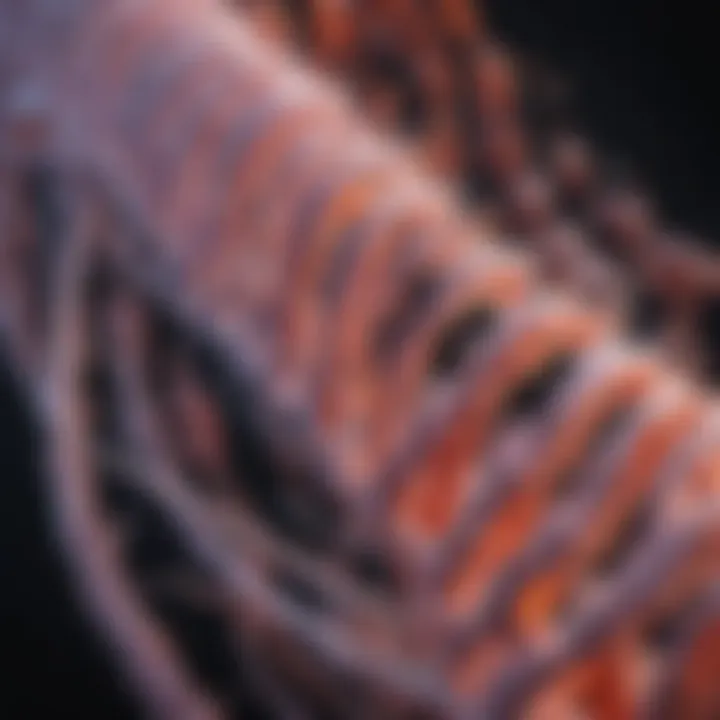
Intro
Protein synthesis is a fundamental biological process that carries immense significance in the realm of molecular biology. At its core, it is the method by which cells create proteins, essential for various cellular functions and biological processes. In the context of AP Biology, understanding protein synthesis involves delving into two key stages: transcription and translation. These processes not only signify how genetic information flows from DNA to functional proteins but also elucidate the intricate mechanisms guiding cellular activities.
As we examine the various steps within transcription and translation, students and educators alike will find it enlightening to grasp how these stages manage to translate the genetic code into tangible molecular structures. Moreover, knowledge of regulatory elements that control these processes plays a crucial role in comprehending the wider implications of protein synthesis. The following sections will explore these concepts, providing clarity and context to the seemingly complex world of molecular mechanisms.
Preface to Protein Synthesis
Protein synthesis is a fundamental biological process that serves as the backbone of life itself. Every organism, from the tiniest single-celled bacterium to complex multicellular mammals, relies on it for growth, repair, and the very function of cells. This article dives into the intricate world of protein synthesis, focusing specifically on the two core processes: transcription and translation.
Definition and Importance
At its core, protein synthesis is the method by which cells construct proteins, which are crucial macromolecules composed of amino acids. These proteins perform a multitude of functions within organisms, such as acting as enzymes, structural components, and signaling molecules.
The significance of understanding protein synthesis cannot be overstated. For students, grasping this topic is crucial for mastering AP Biology, as it encapsulates many other essential concepts, such as gene expression and molecular biology. Moreover, protein synthesis is at the heart of genetic engineering and biotechnology, areas of research that continue to revolutionize medicine and agriculture.
Understanding this process also equips students and researchers to comprehend how malfunctions in protein synthesis can lead to diseases. Misfolded proteins or the absence of key proteins can result in a host of disorders like cystic fibrosis and certain types of cancer. By fully grasping protein synthesis, we not only unlock the secrets of biology but also enhance our ability to tackle complex health issues.
Overview of Synthesis Processes
The process of protein synthesis can be divided primarily into two stages: transcription and translation. Each step is a well-choreographed process reliant on various molecular players that interact seamlessly to ensure the right proteins are produced in the right amounts.
- Transcription occurs in the nucleus and involves the creation of messenger RNA (mRNA) from DNA. This is where the information stored in the DNA is first converted into a form that can be used to produce proteins.
- Translation, which takes place in the cytoplasm, follows transcription. It is the process wherein the mRNA is read by ribosomes, and transfer RNA (tRNA) brings in the corresponding amino acids to form a complete protein.
Together, these processes are often referred to as the "Central Dogma" of molecular biology. Understanding the mechanics of these stages offers profound insight into not just biology but the very principles that govern life.
"Protein synthesis is not merely an academic concept but a gateway to biological understanding that influences health and technology."
In summary, having a solid grasp of protein synthesis paves the way for deeper insights into genetic expression, medical research, and the applications of biotechnology in various fields. Whether you're a student navigating the complexities of AP Biology or a seasoned researcher exploring innovative solutions to biochemical problems, mastering this topic is essential.
The Central Dogma of Molecular Biology
The concept known as the Central Dogma of Molecular Biology is paramount in understanding how genetic information is transformed into the functional molecules that drive life. It outlines a framework that describes the flow of genetic information from DNA to RNA to proteins. This simplified model is not just a theoretical construct; it serves as a practical guide that sheds light on the intricate processes that dictate how traits and functions are expressed in organisms.
Understanding this flow is essential for anyone diving into molecular biology, especially students and researchers grappling with protein synthesis. This dogma stands not only as a theoretical backbone but also illustrates how mutations or intervening factors can disrupt biological systems, potentially leading to diseases or developmental issues.
Understanding DNA, RNA, and Proteins
At the heart of the Central Dogma are three core components: DNA, RNA, and proteins.
- DNA (Deoxyribonucleic Acid) serves as the storage unit for genetic information. It is structured as a double helix composed of nucleotides, each consisting of a sugar, phosphate group, and a nitrogenous base. The sequence of these bases encodes the instructions necessary for building an organism’s proteins.
- RNA (Ribonucleic Acid) plays a crucial intermediary role. It is synthesized during the transcription process from the DNA template and is typically single-stranded. There are several types of RNA, including mRNA (messenger RNA), which carries the code from DNA to the ribosomes where proteins are made, and tRNA (transfer RNA), which brings the appropriate amino acids during translation.
- Proteins are the workhorses of the cell. Comprised of amino acids linked together in specific sequences dictated by mRNA, proteins carry out various functions including structural support, catalyzing biochemical reactions as enzymes, and facilitating communication between cells.
A poignant example illustrating this relationship would be the synthesis of insulin, a critical hormone. The insulin gene, stored in the DNA of pancreatic cells, is transcribed to produce mRNA. This mRNA directs the production of insulin by ribosomes. Understanding this flow of information is fundamental for both students and scientists alike, as it lays the groundwork for more complex genetic and biochemical inquiries.
Interconnection Between Transcription and Translation
The processes of transcription and translation are the two sides of the coin in protein synthesis. They do not operate in isolation but are tightly interwoven, each reliant on the successful completion of the previous step.
- Transcription occurs in the nucleus of eukaryotic cells, where RNA polymerase binds to a promoter sequence on the DNA and unwinds the double helix. It then synthesizes an RNA strand that corresponds to the DNA template.
- Translation, on the other hand, takes place outside the nucleus, within the ribosomes in the cytoplasm. The mRNA generated during transcription serves as a blueprint, guiding the assembly of amino acids into a polypeptide chain, a precursor to a functional protein.
Understanding these processes highlights their dynamic nature. For instance, the efficiency of transcription can impact how much mRNA is available for translation, affecting protein levels within the cell.
As they're not just steps in a workflow but integral parts of cellular communication and function, grasping their relationship allows students and educators to appreciate the elegance of molecular biology and its implications in the grander scheme of life.
"To understand the Central Dogma is like opening a window to see how life itself operates on a molecular level."
Transcription Process
Transcription is a cornerstone of protein synthesis, acting as the initial step where genetic information is copied from DNA to RNA. This process not only sets the stage for translation but also plays a pivotal role in gene expression. The importance of transcription lies in its detailed orchestration; it ensures that the right messages are conveyed at the right times within the cell. Without proper transcription, the entire process of protein synthesis can falter, impacting everything from cellular function to organismal health.
Initiation Phase
Role of RNA Polymerase
The RNA polymerase enzyme is crucial during the initiation phase of transcription. Its primary responsibility is to bind at the promoter region of a gene, unwinding the DNA strands to allow access for the RNA copy to be made. A notable characteristic of RNA polymerase is its ability to synthesize RNA in a 5' to 3' direction, following the template strand of DNA. This directional synthesis is vital, as it directly influences the sequence of nucleotides in the resultant RNA molecule. RNA polymerase, specifically the RNA polymerase II form found in eukaryotes, is a popular focus in this article due to its essential part in transcribing mRNA, which carries the coded instructions from DNA.
One unique feature of RNA polymerase is its ability to initiate transcription without a primer—the enzyme can begin RNA synthesis as soon as it recognizes the proper promoter region. However, this feature can also present drawbacks, as improper binding or recognition of promoter sequences can lead to transcription errors that compromise protein production.
Promoter Regions
Promoter regions are specific sequences of DNA located upstream of a gene, providing binding sites for RNA polymerase. They serve as critical regulatory elements in the transcription process. The key aspect of promoter regions is their diverse design, often containing specific motifs that signal to the RNA polymerase where to start transcribing. This diversity allows for complex regulatory mechanisms, granting the cell flexibility in gene expression.
In this article, the promoter region is emphasized for its role in determining the efficiency of transcriptional initiation. Detecting variations in promoter sequences can offer valuable insights into gene regulation—how, when, and how much a gene is expressed. While the unique combinations of nucleotides in these regions can enhance the precision of gene activation, there can be challenges associated with promoter mutations that lead to misexpression or silencing of vital genes.
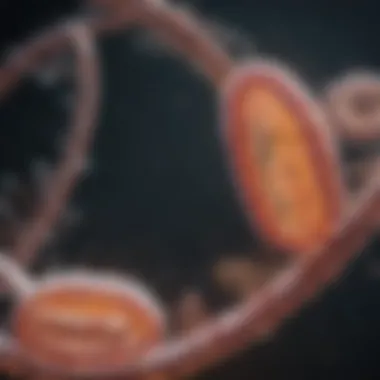
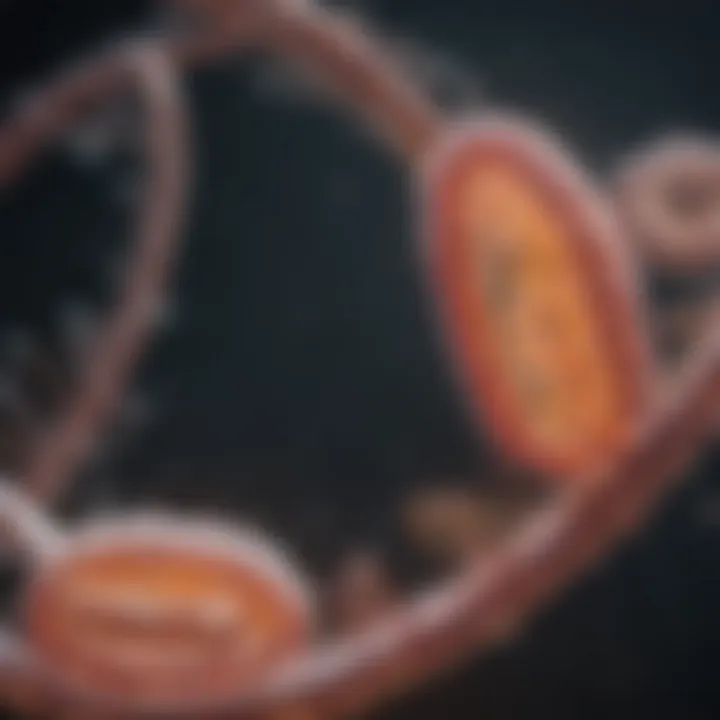
Elongation Phase
RNA Strand Growth
During the elongation phase, the RNA strand is synthesized as RNA polymerase moves along the DNA template strand, adding nucleotides to the growing RNA chain. A key feature of RNA strand growth is its rapid pace; RNA polymerase can add nucleotides at a remarkable rate, which is critical for timely responses in cellular processes. Enhanced RNA strand growth is crucial, especially in cells that need to produce large amounts of protein very quickly.
Although rapid synthesis is beneficial, it also presents challenges. Errors during RNA strand growth can result in faulty transcripts, which may lead to malfunctioning proteins that can disrupt cellular functions. Thus, maintaining fidelity during this process is essential—RNA polymerase has some proofreading capability, but it isn’t as stringent as DNA polymerase.
Nucleotide Pairing Mechanisms
Nucleotide pairing mechanisms dictate how nucleotides are added to the growing RNA strand. The mechanism involves base-pairing with the DNA template–adenine pairs with uracil in RNA, while cytosine pairs with guanine. A significant characteristic of these mechanisms is their reliance on the complementary nature of nucleotides, ensuring accuracy during transcription.
The unique aspect of nucleotide pairing in RNA synthesis allows for variations, such as incorporating specific chemical modifications that can impact RNA stability and functionality. Understanding this pairing not only clarifies how transcription fidelity is maintained but also emphasizes ways that errors can propagate through to the final protein product.
Termination Phase
Termination Signals
Termination signals are sequences in the DNA that signal the end of transcription. These signals can vary, but they often lead to the release of the newly synthesized RNA from the RNA polymerase. A key characteristic of these signals is their ability to halt RNA synthesis accurately, which is vital for proper gene regulation. This article highlights how termination signals not only facilitate the cessation of transcription but also allow the cell to prepare for the next steps in gene expression.
However, these signals can sometimes cause issues, as premature termination or improper signal recognition can lead to truncated transcripts that may not function correctly in downstream processes.
Post-Transcriptional Modifications
Post-transcriptional modifications are processes that occur after the RNA molecule is synthesized, significantly influencing the RNA's stability and functionality. The key characteristic of these modifications is their variety—capping at the 5' end, polyadenylation at the 3' end, and splicing out introns are all critical steps that enhance mRNA quality. This article views these modifications as essential for preparing mRNA for the translation process, ensuring that it is competent for protein synthesis.
These modifications, while largely advantageous, also have caveats. For instance, splice variants can lead to the production of different protein isoforms from a single gene, which adds complexity to gene regulation but is also a source of potential error if not properly managed.
RNA Processing
RNA processing is a critical stage in the journey from gene to protein, ensuring that the RNA formed during transcription is mature and ready for translation. This process is fundamental, not only for the stability of the RNA molecule but also for its functionality in protein synthesis. By refining the initial RNA transcript, cells can effectively regulate which proteins are produced and in what quantity, highlighting the intricate connection between genetic information and phenotypic expression.
Capping and Polyadenylation
One of the first steps in RNA processing is the addition of a 5' cap and a poly(A) tail at the 3' end. The 5' cap, a modified guanine nucleotide, protects the RNA from degradation and helps in the binding of ribosomes during the translation phase. This cap acts as a signal that tells the cellular machinery to recognize the RNA as mRNA instead of other RNA types.
On the other hand, the poly(A) tail, comprised of numerous adenine nucleotides, extends the lifespan of the mRNA in the cytoplasm. Both capping and polyadenylation are essential features that enhance the stability and translatability of mRNA, giving these processes great significance in cellular regulation.
Splicing Mechanisms
After capping and polyadenylation, the next big step involves splicing, which is the process of removing introns and joining together the exons. Introns and exons are key components of the initial RNA transcript: exons are the coding sequences, while introns are non-coding sequences that need to be removed before the RNA can be translated into protein.
Introns and Exons
Introns hold a fascinating place in RNA biology.
- Key Characteristic: Exons carry the information needed for protein synthesis, while introns, often seen as "junk" DNA, may have regulatory roles or contribute to gene evolution.
- Contribution to Goal: Their removal allows the exons to be pieced together, forming a continuous coding sequence. This process of splicing is beneficial as it can lead to alternative splicing, allowing a single gene to produce multiple protein variants. The complexity derived from intron-exon arrangements adds a layer of regulatory control that is essential for diverse protein functions, making it a valuable focus in this discourse.
- Spectra of Organization: Exons can be retained or skipped based on cellular needs, illustrating how dynamic protein synthesis can be.
- Adaptability: This adaptability is key in various biological pathways, showcasing how multicellular organisms can evolve functions.
Spliceosomes
Spliceosomes are the molecular machines that carry out the splicing of pre-mRNA. They are complex structures made up of snRNPs (small nuclear ribonucleoproteins) and numerous proteins that congregate to recognize splice sites.
- Key Characteristic: Spliceosomes are remarkable for their specificity and efficiency. They ensure that the correct sequences are joined, maintaining the integrity of the genetic information.
- Contribution to Goal: This precision is pivotal for ensuring that the proteins produced are functionally accurate and can perform their roles effectively.
- Unique Feature: Spliceosomes are dynamic, able to reconfigure rapidly to process different RNA substrates efficiently. Their ability to carry out precise cuts further emphasizes the importance of this mechanism in the quest for proper gene expression.
- Advantages: This precision ensures that only the necessary coding sequences are preserved while ignoring non-coding regions. Conversely, a malfunction in splicing can lead to serious genetic disorders or cancer, showing that while spliceosomes enhance RNA processing, they also carry the burden of being finely tuned to cellular environments.
"The intrigue of RNA Processing lies not only in the genetic code but also in the elegant nuances that allow for cellular complexity and adaptability."
Thus, understanding the detailed workings of RNA processing — the mechanic roles that capping, polyadenylation, splicing, and the function of spliceosomes play — is essential for a clearer picture of gene expression and its implications in various biological contexts.
Translation Process
The translation process is where the magic truly happens in protein synthesis. This stage is crucial, as it converts the genetic code carried by messenger RNA (mRNA) into a functional protein. Understanding translation not only helps students connect the dots in molecular biology, but it also emphasizes the precision and complexity of life's building blocks. In a sense, translation is like a secret recipe being cooked up in a kitchen, where each ingredient must be just right to yield a successful dish.
Ribosome Structure and Function
Ribosomes, often referred to as the cell's "protein factories," are paramount in the translation process. These small structures are made up of ribosomal RNA (rRNA) and proteins, and they can either float freely in the cytoplasm or be attached to the endoplasmic reticulum. Their primary function is to read the mRNA sequence and orchestrate the assembly of amino acids into polypeptides.
Ribosomes also hold three critical sites: the A site, P site, and E site. The A site is where the tRNA carrying the next amino acid arrives, the P site holds the tRNA with the growing polypeptide chain, and the E site is where the tRNA exits once it has delivered its amino acid. This spatial arrangement is not just convenient; it's essential for the efficiency and accuracy of protein synthesis.
Initiation of Translation
The initiation phase kicks off the translation process with a series of intricate steps. First, the ribosome assembles around the start codon on the mRNA, which is typically AUG. This codon not only signals where translation begins but also encodes for the amino acid methionine, making it a critical starting point.
Start Codon Recognition
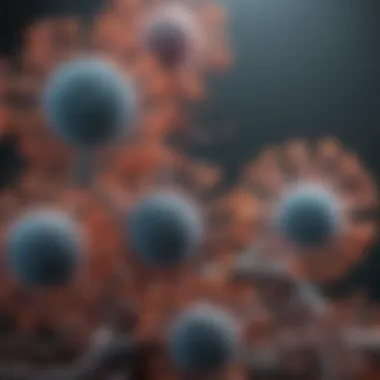
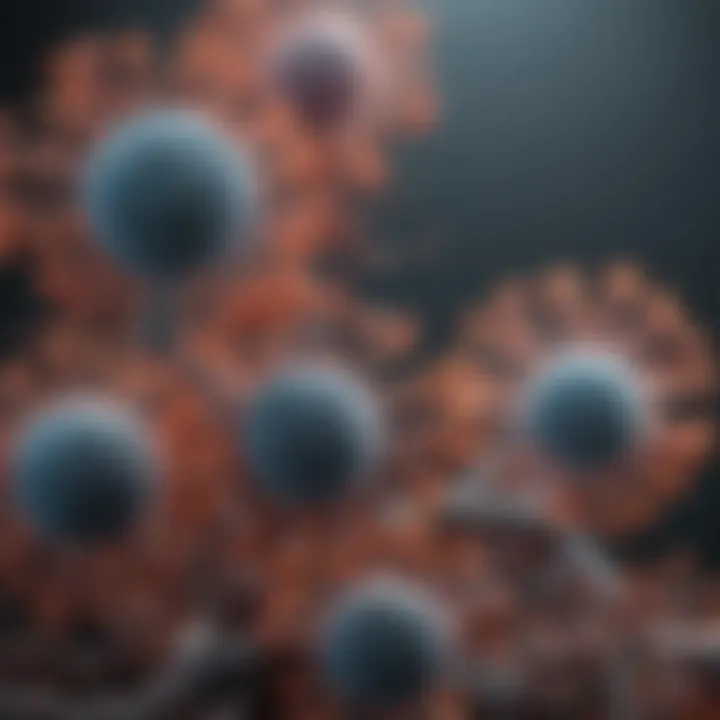
Start codon recognition is a vital step that ensures the ribosome correctly identifies where to begin translating the mRNA into protein. The presence of the AUG codon is what directs the ribosome to initiate the process at the right place. This characteristic of AUG sets it apart, establishing it as a consistent and reliable starting point for protein synthesis.
Without this recognition, ribosomes could lose their way, potentially leading to dysfunctional proteins. It's a beneficial choice in this article because it highlights the precision inherent in genetic translation. The unique feature of AUG is its dual role as a start signal and as a coding mechanism for methionine, which is fundamental for protein synthesis.
tRNA Activation
On the subject of tRNA activation, this process adds another layer of complexity to translation. Each tRNA molecule is charged with its corresponding amino acid by an enzyme known as aminoacyl-tRNA synthetase. This step is critical because the tRNA must carry the correct amino acid that matches the mRNA codon.
The noteworthy aspect of tRNA activation is its specificity; each amino acid has a corresponding tRNA that must pair correctly to ensure fidelity in protein synthesis. This makes it an essential feature of translation, as any errors in this step can lead to the production of malfunctioning proteins. What sets this apart in our discussion is that it represents a meticulous mechanism that organisms use to maintain accuracy in their biochemical processes.
Elongation and Termination
Elongation and termination are the final stages of translation, allowing the ribosome to construct the polypeptide chain effectively.
Peptide Bond Formation
Peptide bond formation is a critical chemical reaction that links amino acids together, forming the long chains that ultimately fold into proteins. During this process, the ribosomal machinery catalyzes the bond between the amino group of one amino acid and the carboxyl group of another.
This unique feature of peptide bond formation comes with a structural characteristic: the resulting bond is planar and has partial double bond character, which grants the polypeptide chain its rigidity. In this article, focusing on this step highlights how intricately nature has designed the machinery of life, ensuring that proteins are assembled correctly while minimizing errors.
Stop Codon Function
Finally, the stop codon function concludes the translation process. Stop codons—UAA, UAG, and UGA—signal the ribosome to cease translation, ultimately releasing the completed polypeptide. This completion is necessary for the maintenance of the cell’s physiology, as unregulated protein synthesis would lead to chaos within the cell.
The character of stop codons is significant because they ensure that proteins are synthesized only when necessary. Their unique property is the ability to be recognized by release factors, which lead to the disassembly of the translation complex. This makes stop codons essential not just for ending the process, but for regulating protein levels within the cell. Their role in our discussion is to showcase how translation processes are not just mechanical; they are also regulated pathways that maintain cellular homeostasis.
Post-Translational Modifications
Post-translational modifications (PTMs) are critical adjustments that proteins undergo after their initial synthesis. These modifications are not merely decorative; they fundamentally influence how proteins function in diverse biological contexts. For students engaged in AP Biology, understanding PTMs is essential for grasping the complex nature of protein functionalities and interactions within cellular mechanisms.
The process of protein synthesis doesn’t end once translation wraps up. Instead, proteins often require additional tweaks to ensure they perform their specific roles effectively. The benefits of these modifications include enhanced protein stability, localization, and signaling capabilities. Neglecting to consider PTMs could lead to a misinterpretation of how proteins operate, ultimately oversimplifying the intricate biology at play.
"Post-translational modifications can dramatically change the role or activity of a protein, highlighting that the journey of a protein extends well beyond its translation."
Types of Modifications
Phosphorylation
Phosphorylation involves the addition of a phosphate group to a protein. This modification can serve as a light switch, turning protein activities on or off in response to various signals. It's highly versatile, affecting processes like cell division, metabolism, and apoptosis.
One key characteristic of phosphorylation is its rapid reversibility. Enzymes such as kinases add phosphate groups, while phosphatases remove them. This dynamic regulation allows cells to quickly adapt to changing environments. Moreover, phosphorylation has become a prevalent focus in studies regarding signal transduction, making it a central theme in molecular biology.
However, while phosphorylation offers significant advantages in modulating protein functions, excessive or incorrect phosphorylation can lead to problems. For instance, deregulation is commonly observed in many cancers, indicating that maintaining the balance is crucial for cellular health and proper function.
Glycosylation
Glycosylation refers to the addition of sugar molecules to proteins, influencing their structure and function. This modification is particularly essential for proteins destined for secretion or those that reside on cell surfaces. It contributes to stability and helps proteins avoid proteolytic degradation, prolonging their lifespan in the biological sphere.
A key aspect of glycosylation is its variety. There are numerous types of glycosylation, such as N-linked and O-linked, each serving distinct roles in cellular processes. These unique features enable glycosylated proteins to be recognized by other molecules, playing roles in signaling, immune response, and even pathogen recognition.
However, the complexity of glycosylation can also pose disadvantages. The various possible combinations of sugars can lead to heterogeneous populations of glycosylated proteins, complicating their study and application in biotechnology.
Role in Protein Functionality
The role of post-translational modifications in protein functionality cannot be overstated. PTMs affect how proteins fold, interact, and are degraded, directly influencing their biological roles. They act as molecular markers, guiding proteins to their proper locations within the cell and determining interaction networks that are crucial for cellular communication.
Regulatory Mechanisms in Protein Synthesis
Regulatory mechanisms are the unsung heroes of protein synthesis. They orchestrate the delicate balance of gene expression, ensuring that proteins are synthesized at the right time and in the right amounts. This balance is crucial for the cell's proper functioning and response to various internal and external stimuli. Without these mechanisms, there could be chaos; proteins might be overproduced or underproduced which can lead to significant biological implications, including diseases.
Gene Regulation Overview
Gene regulation is a process that controls the timing and rate at which genes are expressed and, consequently, which proteins are produced. This process involves several layers and strategies. A vital point to understand is that not all genes are active all the time; cells have the remarkable ability to turn genes on or off depending on what they need. This ability makes gene regulation the conductor in the symphony of protein synthesis.
- Types of Gene Regulation: Gene regulation can occur at several stages, including:
- Importance of Regulation: Without effective regulation, organisms might face issues like metabolic imbalances or developmental defects. For example, in human health, the misregulation of gene expression can lead to cancer, where the production of proteins that drive cell division is uncontrollably activated.
- Transcriptional Level: The most common level of regulation. This involves influences on the initiation of the transcription process, determining whether a gene is transcribed into RNA.
- Post-Transcriptional Level: After transcription, the mRNA can be spliced, altered, or degraded which also affects how much protein is produced.
- Translational Level: This controls the efficiency and rate of translation, thereby impacting how much protein is synthesized from the mRNA.
Transcription Factors
Transcription factors are the key players in the mechanism of gene regulation. These proteins bind to specific DNA sequences, influencing the transcription of particular genes. Consider them as the traffic lights of gene expression; they tell the cellular machinery when to stop or go.
- Types of Transcription Factors: They can be broadly categorized into:
- Mechanisms of Action: These factors often interact with each other and with additional proteins to form complexes that either promote or hinder transcription. It's akin to a dance, where each participant must know their role and timing to create a harmonious outcome.
- Activators: They enhance the transcription of specific genes by helping RNA polymerase to bind to the promoter region more effectively.
- Repressors: In contrast, repressors inhibit transcription by blocking RNA polymerase's access to the gene or competing with activators.
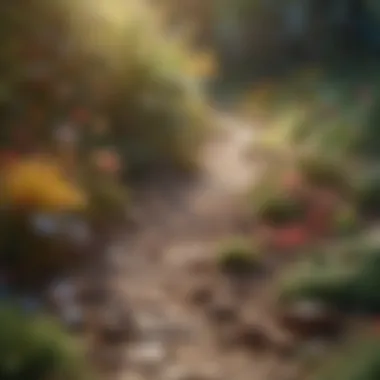
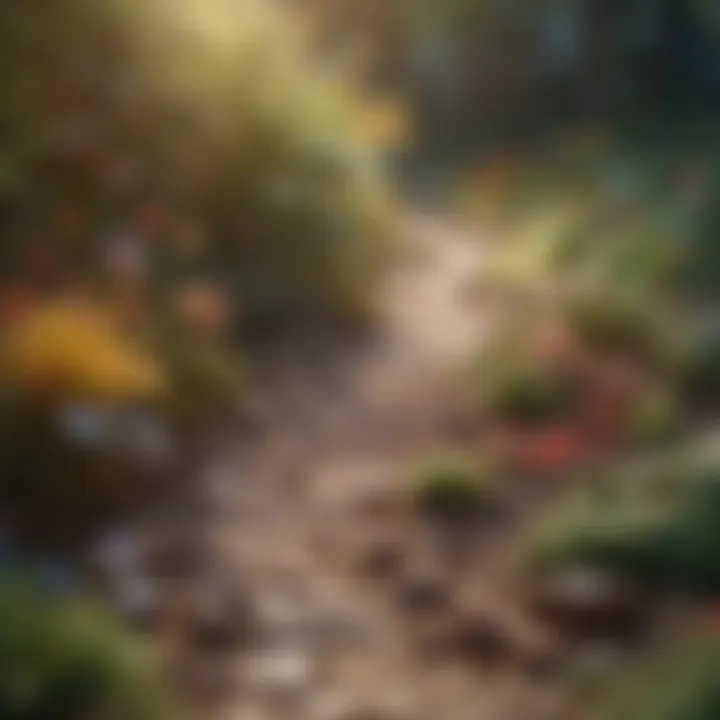
"Transcription factors are like conductors of an orchestra, guiding the different instruments to play in harmony during gene expression."
- Environmental Influence: External signals can also affect the activity of transcription factors. For example, stress hormones can alter their activity, prompting an immediate change in protein production to help the organism adapt.
Understanding these regulatory mechanisms positions one to appreciate the complexity and sophistication of biological processes. The interplay between transcription factors and gene regulation not only governs immediate cellular responses but also shapes the long-term adaptability of organisms, illustrating the profound impact that regulatory mechanisms have on life.
Challenges in Understanding Protein Synthesis
Understanding the mechanisms of protein synthesis presents a range of challenges for students diving into AP Biology. First and foremost, this topic is pivotal since protein synthesis is at the core of cellular functions. Grasping the subtle nuances of transcription and translation not only builds a solid foundation for further biological study but also enhances comprehension of related fields, such as genetics and biotechnology. However, these complex processes can seem overwhelming.
Common Misconceptions
One of the key hurdles in mastering protein synthesis is the prevalence of misconceptions. Many students often mistakenly believe that transcription and translation occur as isolated events, when in fact, they are interconnected processes that flow seamlessly into one another.
Another prevalent misunderstanding is about the roles of RNA. It's not just a messenger but also plays multiple vital roles. For instance, students might think that RNA serves only as a temporary copy of DNA, neglecting its importance as a functional molecule in ribosomes or as a translator in tRNA. This limited view can obscure larger biological concepts, such as gene regulation and mutation consequences.
Additionally, students might confuse the functions of different types of RNA. For example:
- mRNA carries the blueprint from DNA to ribosomes.
- tRNA brings the correct amino acids to the ribosome for protein assembly.
- rRNA forms the scaffold for ribosomal structure, enabling protein synthesis.
Such distinctions are crucial, yet often overlooked, leading to gaps in understanding.
"Misunderstanding protein synthesis processes can leave students feeling lost, undermining their confidence in biological sciences."
Complexities of Molecular Processes
Yet, misconceptions are just one part of the puzzle. The molecular biology of protein synthesis is inherently complex. Students must grapple with various technical details that often seem abstract. For instance, the various phases of transcription—initiation, elongation, and termination—require an appreciation of the biochemical interactions involved.
During translation, students encounter a parallel complexity. The ribosome's function isn't merely to bind molecules together; it involves a fine-tuned orchestration of numerous components. From codons to anticodons, this intricate dance of nucleotides must align properly for accurate protein formation.
Furthermore, the introduction of molecular signals that regulate these processes means that students also have to comprehend various feedback mechanisms and their implications on genetic expression. Learning about post-translational modifications adds another layer, revealing how proteins can be altered after synthesis to refine their functionality.
- Phosphorylation can activate or deactivate many proteins, influencing their roles significantly.
- Glycosylation affects protein stability and recognition, demonstrating the diverse roles post-translational modifications play.
The difficulties in understanding these layers of complexity stem from their interdependent nature. Students must learn to navigate through not just the “how” of these processes, but also the “why” behind the variations and regulations that govern protein synthesis.
In summary, tackling the challenges associated with understanding protein synthesis requires deliberate focus on correcting misconceptions and embracing the complexities that define molecular processes. As learners engage deeper with these topics, they equip themselves with the necessary tools to dissect and appreciate the intricate world of protein synthesis.
Applications and Implications of Protein Synthesis Study
Understanding protein synthesis is crucial in various scientific fields, including biotechnology, medicine, and molecular biology. Recognizing how proteins are built helps illuminate many biological functions and processes that govern life itself. This knowledge is particularly relevant for students, educators, and professionals aiming to grasp both fundamental and advanced concepts relating to biology.
Protein synthesis is at the heart of biological functions, influencing everything from cellular structure to the immune response. Moreover, the insights gained through studying this topic lead to practical applications that extend beyond academia, affecting industries like healthcare, agriculture, and environmental science.
Biotechnology and Genetic Engineering
In the realm of biotechnology, the understanding of protein synthesis paves the way for genetic engineering. Techniques such as CRISPR-Cas9 and recombinant DNA technology rely heavily on manipulating the mechanisms of transcription and translation.
- Gene Cloning: By inserting foreign genes into plasmids, scientists can create proteins that have desired traits, such as increased crop yield or resistance to pests. This is done by harnessing the host cells' machinery for transcription and translation, effectively turning them into factories for the proteins.
- Therapeutic Proteins: Many biopharmaceuticals, like insulin or monoclonal antibodies, are produced through biotechnological methods that utilize engineered cells. Understanding the intricacies of how proteins are synthesized enables researchers to refine these processes to produce drugs more effectively. The ability to produce these proteins accurately is vital for effective treatment options in medicine.
Furthermore, genetic engineering techniques, founded on our understanding of protein synthesis, enable gene therapies aimed at correcting genetic disorders by modifying the expression of faulty genes.
Understanding Disease Mechanisms
Diving deeper into understanding how proteins are made can also shed light on various disease mechanisms. Many diseases stem from problems in protein synthesis, whether from mutations in the DNA that affect codons or errors in RNA processing.
- Genetic Disorders: Conditions like cystic fibrosis result from a malfunction in the protein synthesis process. Understanding how the proteins are made and modified can lead to better treatments and therapies. For example, therapies that can potentially correct or compensate for the faulty protein production are based on detailed knowledge of transcription and translation.
- Cancer Research: Abnormalities in protein synthesis pathways often play a significant role in cancer development. Targeting specific proteins involved in the cell cycle can offer avenues for treatment, signaling the importance of understanding these synthesis pathways.
"The more we delve into the details of protein synthesis, the closer we get to unraveling the complexities of cellular function and disease pathology."
Thus, advancements in treatments and therapies owe much to the foundational knowledge gained through studying protein synthesis. Relevantly, educators leverage these applications to help students appreciate the real-world implications of their studies, fostering a more nuanced understanding of biology.
Finale
When it comes to comprehending protein synthesis, looking at the conclusion is like finding yourself at the finish line of a marathon. It encapsulates the entire journey through transcription and translation, reminding us of the monumental importance of these processes in the broader scope of cellular function and genetic expression.
Summary of Key Points
In reflecting on the key points discussed, we can pinpoint several important aspects:
- Process Overview: Protein synthesis is fundamentally divided into two critical stages: transcription, where the DNA sequence is converted into mRNA, and translation, where the mRNA is interpreted to assemble amino acids into proteins.
- Role of Enzymes: Key players such as RNA polymerase in transcription and ribosomes in translation showcase the intricate teamwork within the cell, ensuring precise execution of protein synthesis.
- Importance of Modifications: Post-transcriptional and post-translational modifications significantly impact protein function, indicating that synthesis is just the first half of the story.
- Regulatory Mechanisms: Gene regulation is an essential aspect of protein synthesis, ensuring that proteins are produced in response to cellular needs, thus allowing for adaptability and survival of the organism.
Taken together, these points highlight not just the mechanics of the process but also the dynamic nature of cellular biology.
Future Directions in Study
Looking ahead, several future directions in the study of protein synthesis offer exciting avenues for exploration:
- Advancements in Biotechnology: As researchers delve deeper into protein synthesis, innovations in biotechnology may lead to more targeted genetic engineering applications, potentially revolutionizing medicine and agriculture.
- Understanding Genetic Disorders: Further research may unveil more about how disruptions in the synthesis process can lead to diseases, fostering advancements in therapeutic strategies.
- Emerging Technologies: With techniques like CRISPR and high-throughput sequencing gaining momentum, the understanding of gene expression and the intricacies of protein synthesis could reach new heights.
In essence, as we tie up this discussion, recognizing the nuances and future directions in protein synthesis can inspire students, educators, and researchers alike to keep pushing the boundaries of our understanding in this vital area of biology. The landscape of protein synthesis is anything but static; it's an ever-evolving field ripe with possibilities.