Understanding Quantum Yield: Key Concepts and Applications
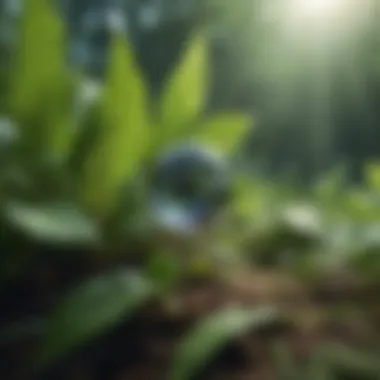
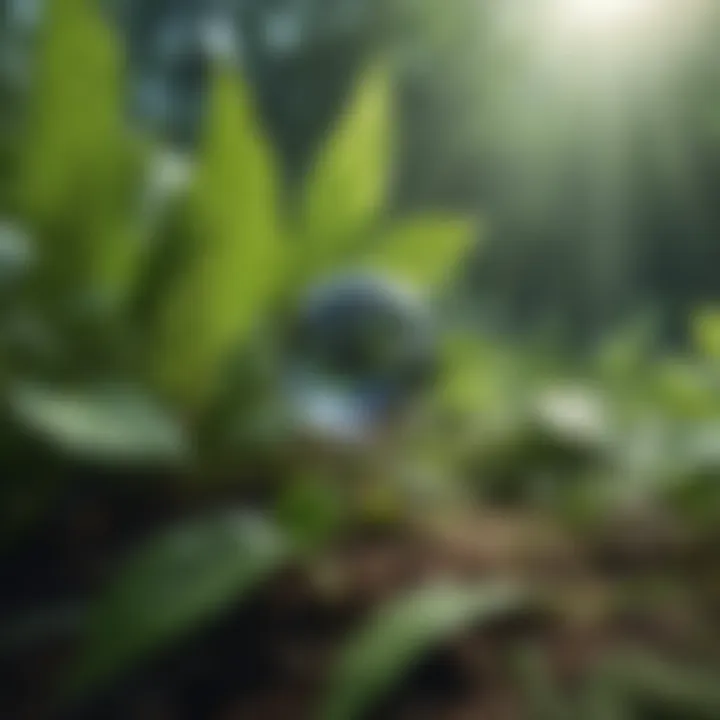
Intro
Quantum yield is a pivotal concept across various scientific disciplines, including chemistry, physics, and biology. It provides insight into the efficiency of a photophysical process or reaction, defined as the ratio of the number of events occurring to the number of photons absorbed. Understanding this concept is essential for a range of applications from photosynthesis to fluorescence, shaping both experimental methodologies and theoretical frameworks.
This article will explore the nuances of quantum yield, emphasizing its measurement techniques and the factors influencing it. By delving into its significance in processes like photochemistry and biological functions, we aim to elucidate the implications of quantum yield for scientific research and technological advancements. A clear understanding of this topic can enrich the knowledge of students, educators, and professionals alike; thus, fostering a broader appreciation of its applications.
Research Highlights
Overview of Key Findings
Quantum yield is not just a theoretical construct; it influences key processes in nature and technology. Here are the important aspects:
- Definition and Importance: Quantum yield defines the efficiency of a light-induced process and plays a key role in fields such as solar energy and biological light reactions.
- Measurement Techniques: Several methods exist for determining quantum yield, including direct measurements in controlled environments and mathematical calculations.
- Factors Influencing Quantum Yield: Environmental conditions, the nature of the absorbing material, and the wavelength of incident light significantly affect quantum yield.
Significance of the Research
The research surrounding quantum yield is vital for numerous reasons:
- It offers insights into improving the efficiency of solar cells through enhanced light absorption.
- Insights from quantum yield studies can inform the development of sustainable energy sources, reflecting the urgent need for environmentally friendly technologies.
Prolusion to Quantum Yield
Quantum yield is a pivotal concept in the realms of chemistry, physics, and biology. Understanding it can significantly enhance our grasp of various fundamental and applied scientific processes. Quantum yield is particularly crucial for interpreting phenomena such as photosynthesis, photochemistry, and fluorescence. By participating in the dialogue of energy transfer, quantum yield offers researchers insights into efficiency and effectiveness in these processes.
In this section, we will explore the definition and historical context of quantum yield. A clear definition establishes a foundational understanding, which is paramount for engaging with more complex applications and implications of this concept. Furthermore, examining its historical development provides context that can deepen appreciation for its current significance.
Definition of Quantum Yield
Quantum yield is defined as the ratio of the number of events of interest to the number of photons absorbed. In simpler terms, it measures the efficiency of a photon-induced process. A quantum yield of 1 denotes that every absorbed photon results in an event, while a yield less than 1 indicates that not all absorbed photons lead to the expected outcomes. Conversely, yields greater than 1 suggest additional processes enhancing the event rate, which can occur in specific molecular conditions.
This measurement is critical in evaluating processes from chemical reactions to biological systems. Each application may have its unique interpretation of quantum yield, thus necessitating a closer examination in subsequent sections.
Historical Context
The comprehension of quantum yield has evolved significantly since its inception. Its roots trace back to the early 20th century when scientists began investigating the interactions between light and matter. Initial studies focused on basic photochemical reactions. Over time, advancements in instrumental technology provided clearer insights into these processes, enabling researchers to attribute specific behaviors to quantum yield.
One pivotal moment in this development came with the introduction of the concept in photochemistry by researchers such as Martin Gouterman and Gratzel, who provided frameworks for understanding various energy transfers.
As the field of quantum yield continued to grow, researchers began applying the concept across interdisciplinary boundaries. This ongoing evolution highlights the importance of quantum yield across multiple scientific domains, revealing connections that were not previously recognized.
Mathematical Representation
Mathematical representation of quantum yield forms the backbone of quantitative analysis, providing a clear framework for understanding the efficiency of photochemical processes. This section will detail the fundamental aspects of how quantum yield is expressed mathematically, its benefits, and crucial considerations.
Quantitative Analysis
In the realm of physical sciences, the term quantum yield refers to the efficiency with which absorbed light produces a particular outcome, often related to chemical reactions or fluorescence. It quantifies this efficiency by expressing the ratio of the number of photons emitted to the number of photons absorbed. Mathematically, this is often represented as:
Where:
- ( \Phi ) symbolizes the quantum yield,
- ( N_emitted ) denotes emitted photons,
- ( N_absorbed ) refers to absorbed photons.
This simple yet effective formula encapsulates a rather intricate interplay of variables that define the photophysical performance of a material or system. Understanding these quantitative measures can guide research in optimizing numerous applications, ranging from solar energy conversion to biomedical imaging.
Units of Measurement
The units of measurement for quantum yield can often create confusion. Because quantum yield is a ratio, it is dimensionless; however, understanding the related measurements remains relevant.
To contextualize, we often encounter quantum yield values expressed in specific scenarios, such as:
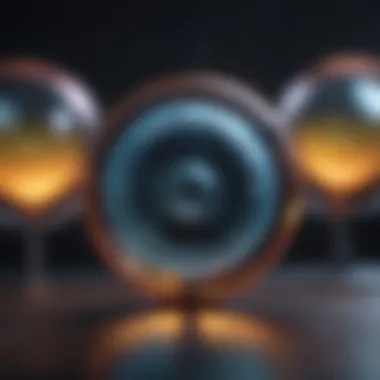
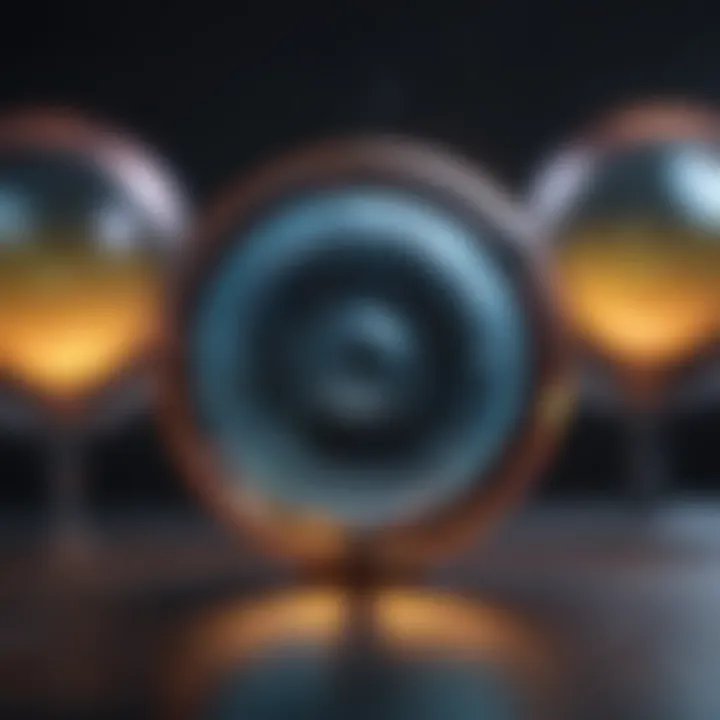
- Photosynthesis: Tied to chlorophyll efficiency, yielding values that indicate how well plants convert light into chemical energy.
- Fluorescence: Indicative of the efficiency of a fluorescent dye or marker, relevant in imaging and bioassays.
In research contexts, quantum yield is pivotal for comparing the performance of various molecules under different experimental conditions. Variability in results often stems from differences in measurement techniques or environmental settings, underlining the importance of adhering to standardized protocols. Overall, precise measurement practices enhance the reliability of data, enabling vibrant discussions in the scientific community.
To summarize, a profound comprehension of the mathematical representation and quantitative analysis of quantum yield illuminates its fundamental role in scientific inquiry. As we explore further, the complexity of the factors influences how quantum yield manifests across varied applications.
Measurement Techniques
Measurement techniques are crucial for quantifying quantum yield, an essential concept in various scientific fields. Accurate measurement of quantum yield facilitates understanding of how effectively a system converts absorbed light into useful energy. It also informs researchers about the efficacy of materials in applications such as solar cells and fluorescence imaging. Mastering these techniques can significantly impact both practical applications and theoretical research.
Direct Measurement Methods
Direct measurement methods provide a straightforward approach to determining quantum yield. These techniques typically involve measuring the intensity of emitted light from samples exposed to specific wavelengths of excitation light. Photoluminescence spectroscopy is one such method. In this technique, a sample absorbs light which then leads to the emission of photons. By comparing the emitted light's intensity to the intensity of the absorbed light, one can calculate the quantum yield.
Another direct method includes the use of pulsed laser systems that allow precise timing of excitation and emission events. This precision helps in minimizing errors in measurements. Notably, the advantages of direct methods include higher accuracy and real-time data collection. However, careful consideration must be given to factors such as sample concentration and environmental stability, as they can affect measurement outcomes significantly.
Indirect Measurement Techniques
Indirect measurement techniques offer alternative approaches to calculating quantum yield. These methods often involve comparison with reference standards known to have specific quantum yields. One common technique is referred to as the comparison method. In this method, the quantum yield of an unknown sample is determined by comparing it to a standard sample with a known value.
These techniques provide flexibility and can be used when direct measurements might be challenging or impractical. However, one must approach these techniques with caution; interferences from the sample matrix can skew results. Additionally, the choice of the reference standard is critical. An inaccurate reference can result in miscalculated quantum yields, possibly leading scientists astray in their research. Therefore, it is necessary to validate indirect measurements carefully.
Instrumentation Used
Instrumentation plays a significant role in the accurate measurement of quantum yield. Some key instruments include spectrophotometers and fluorometers. A spectrophotometer measures the absorption spectrum, which is essential for determining the amount of light absorbed by a sample. The data obtained from this instrument forms the baseline for calculating quantum yield.
Fluorometers specifically measure fluorescence, collecting data on emitted light. Using photomultiplier tubes or CCD cameras, these instruments can assess emitted light's intensity and timing with great precision. To further enhance measurement accuracy, instrumentation often incorporates filters that isolate specific wavelengths, reducing background noise that can interfere with data quality.
Factors Influencing Quantum Yield
Understanding the factors that influence quantum yield is essential for a nuanced comprehension of its applications. Quantum yield can vary significantly based on a range of elements. These factors provide insight into both the efficiency of photophysical processes and the conditions under which they occur. This section will delve into two primary influences: environmental conditions and the chemical environment.
Environmental Conditions
Environmental conditions play a pivotal role in determining quantum yield. Factors such as temperature, light intensity, and the presence of solvents or other materials can greatly affect how a system absorbs and emits energy.
- Temperature: Temperature impacts the kinetic energy of molecules, which can influence reaction rates and efficiencies. Higher temperatures can increase molecular motion, potentially leading to non-radiative decay pathways that decrease quantum yield.
- Light Intensity: The intensity of light sources also dictates the level of excitation in a sample. Insufficient light may lead to lower excitation rates, while excessive light could increase photobleaching, which compromises the quantum yield.
- Solvent Effects: Solvents have unique properties that can alter the photophysical outcomes of substances. Polar solvents might stabilize certain excited states, while non-polar solvents could do the opposite, impacting overall quantum yield.
Navigating these environmental conditions is critical for researchers. A well-controlled environment will lead to more reliable measurements of quantum yield, ultimately fostering a deeper understanding of the underlying processes.
Chemical Environment
The chemical environment in which a reaction takes place is equally vital to quantum yield. The identity and concentration of reactants, as well as the presence of catalysts or inhibitors, can shift quantum yields considerably.
- Reactant Interaction: Different reactants can exhibit distinct interactions with light. For example, molecular structure can determine how a substance behaves under light exposure, influencing its emission and absorption properties.
- Concentration Levels: High concentrations may lead to self-absorption phenomena, where emitted light is re-absorbed by other molecules, subsequently lowering the detected quantum yield. Balancing concentration is essential in experimental setups.
- Presence of Catalysts: Catalysts can facilitate reactions, potentially increasing quantum yield by creating favorable conditions for energy transfer and emission.
A thorough understanding of both the environmental and chemical factors is crucial for optimizing experiments and applications involving quantum yield. Research in this area continues to evolve, revealing more intricate relationships that govern these processes.
"Understanding the variables affecting quantum yield can inspire innovative advancements in numerous scientific fields."
In summary, the interplay of environmental and chemical variables significantly informs quantum yield outcomes. Carefully evaluating these factors helps to improve experimental design and enables deeper insights into photophysical phenomena.
Applications of Quantum Yield
Quantum yield is a fundamental concept that has substantial implications across various scientific fields. Its applications are not limited to theoretical constructs; they play a crucial role in understanding and improving processes that are vital to both nature and technology. This section aims to elucidate the significance of quantum yield, specifically how it impacts areas like photosynthesis, photochemistry, and fluorescence. Understanding these applications helps to grasp not just the concept itself, but also its broader relevance in scientific research and practical applications.
In Photosynthesis
In the world of biology, quantum yield is particularly significant in photosynthesis. This process involves converting light energy into chemical energy, primarily in plants, algae, and some bacteria. The quantum yield of photosynthesis refers to the efficiency with which absorbed light is converted into chemical energy. It is a critical factor influencing plant growth and productivity.
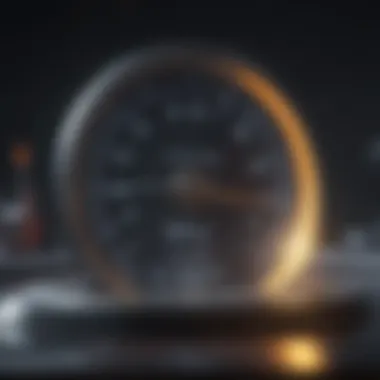
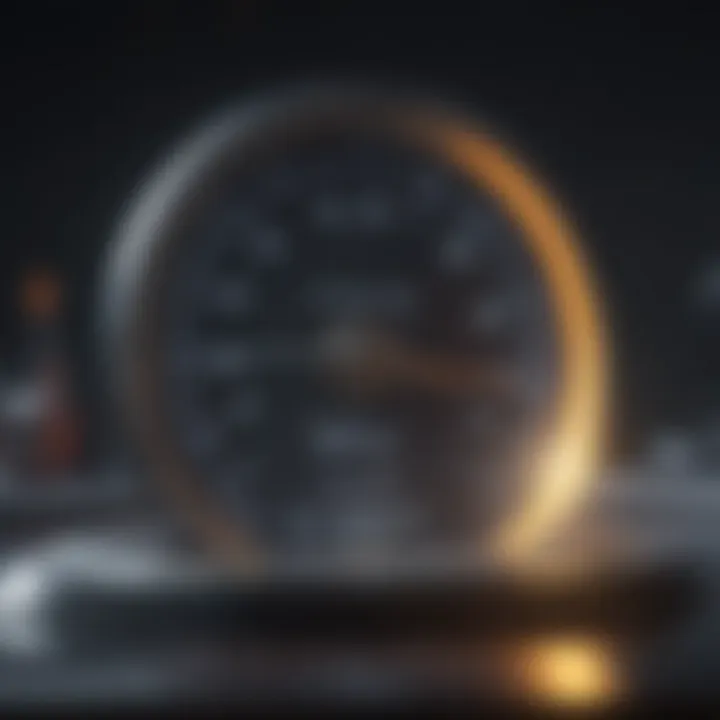
Optimizing the quantum yield can lead to improved agricultural practices. By increasing the efficiency of photosynthesis, crops can produce more biomass and yield, which is increasingly important given the growing global population. Some studies suggest that interventions such as controlled lighting conditions can considerably affect the quantum yield of different plant types.
In Photochemistry
Photochemistry, the study of chemical reactions initiated by light, heavily relies on the concept of quantum yield. In photochemical reactions, the quantum yield indicates the efficiency with which absorbed photons lead to the desired product formation. Higher quantum yields signify that more reactants convert into products, making these reactions more economically viable.
An example of its application is in the production of solar fuels. Understanding and improving the quantum yield of photochemical reactions can enhance the efficiency of converting solar energy into chemical energy, which is a promising avenue for sustainable energy solutions. This knowledge allows researchers to design more effective catalysts and improve the overall energy conversion processes.
In Fluorescence and Imaging Techniques
Fluorescence involves the absorption of light by a substance and the subsequent emission of light, often used in various imaging techniques like fluorescence microscopy. Quantum yield plays a key role in these applications as it determines the brightness of the fluorescent signal. A higher quantum yield results in more intense fluorescence, which enhances the resolution and clarity of imaging techniques.
In medical diagnostics, understanding fluorescence quantum yield assists in developing better imaging agents. For instance, researchers have utilized quantum yield data to improve the performance of fluorescent probes that can detect specific tissues or disease markers. This has significant implications in early disease detection and biomedical research, offering a powerful tool for scientists and clinicians alike.
"The quantum yield acts as a bridge between light and chemical action in scientific applications, influencing efficiency in processes related to energy conversion and imaging."
Quantum Yield in Biological Systems
Quantum yield represents a crucial aspect of biological systems, particularly in how they convert energy. This section explores the role of quantum yield in bioluminescence and its implications for cellular processes. Understanding these facets not only enhances our comprehension of life at the cellular level but also opens pathways for research and applications in various scientific fields.
Role in Bioluminescence
Bioluminescence is a fascinating phenomenon where living organisms produce light. This process is visible in certain species, such as fireflies and deep-sea creatures. The quantum yield here indicates the efficiency of light production when energy from biochemical reactions is converted into photons. A high quantum yield means that a significant portion of the absorbed energy is transformed into light.
The efficiency of bioluminescence holds ecological importance. It aids in attracting mates, deterring predators, or luring prey. For instance, the firefly uses its light to attract mates during reproductive seasons. The effectiveness of this light signal is directly related to the quantum yield. Lower efficiencies may result in fainter signals, potentially affecting survival and reproduction.
Implications for Cellular Processes
Quantum yield also has broader implications for cellular processes. Cellular respiration, photosynthesis, and signal transduction pathways are some areas where quantum yield plays a vital role. In photosynthesis, for instance, the efficiency of the light reactions is measured by its quantum yield. A higher quantum yield suggests that plants can convert light energy into chemical energy more efficiently, benefiting growth rates and biomass production.
Likewise, in cellular respiration, quantum yield influences how energy from nutrients is utilized. The efficiency of ATP synthesis during these processes can be linked back to quantum yield, impacting cell metabolism and overall energy balance.
In summary:
- Quantum yield in bioluminescence impacts communication and survival strategies within ecosystems.
- In cellular processes, a higher quantum yield enhances energy conversions, leading to improved growth and metabolism.
"The study of quantum yield in biological systems not only enhances theoretical knowledge but also holds practical importance in biotechnology and environmental sciences."
Developing a deeper understanding of these relationships can fuel innovations in fields such as bioengineering and ecological conservation. As research into quantum yield continues to evolve, it serves as a bridge connecting fundamental biological concepts to applied science.
Emerging Trends and Research
The examination of emerging trends and research in quantum yield represents a critical juncture in our understanding of this concept. As new methodologies develop, they enhance the measuring capabilities of quantum yield, which consequently benefits diverse fields ranging from nanotechnology to environmental science. Addressing the latest advancements in quantum yield measurement and its applications opens avenues to refine scientific processes and explore novel innovations.
Advancements in Quantum Yield Measurement
Recent technological advancements in measuring quantum yield are substantial. The integration of advanced spectral techniques allows for more precise recordings of photons emitted versus absorbed. Time-resolved spectroscopy has emerged as a potent tool, granting insights into the kinetics of excited states and assisting in determining quantum efficiency with greater accuracy.
"Innovations in measurement technology are reshaping how we comprehend and apply quantum yield across disciplines."
New computational methods also enhance the interpretation of experimental data. Simulation software can now predict quantum yields based on molecular structure, which streamlines experimental design. This approach enables researchers to focus on promising candidates, thus expediting the development process in chemical and biological contexts.
Overall, these advancements are essential for amplifying our understanding of how different parameters influence quantum yield, allowing for a more tailored application in real-world scenarios.
Quantum Yield in Nanotechnology
Quantum yield plays a pivotal role in nanotechnology, particularly in the development of nanomaterials used for applications in electronics and biomedical fields. The unique optical properties of nanoparticles often correlate with high quantum yields, making them valuable in areas such as imaging and sensing.
Consider the application of quantum dots in solar cells. Their high quantum yields enhance energy conversion efficiency, directly impacting the effectiveness of photovoltaic devices. This relates to key applications in sustainable energy, where maximizing output is a primary goal.
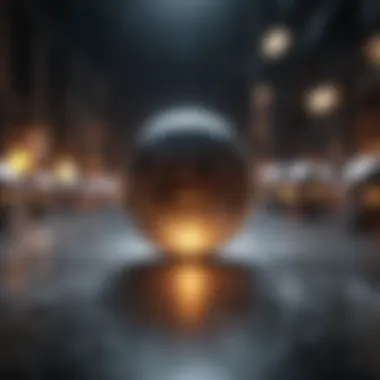
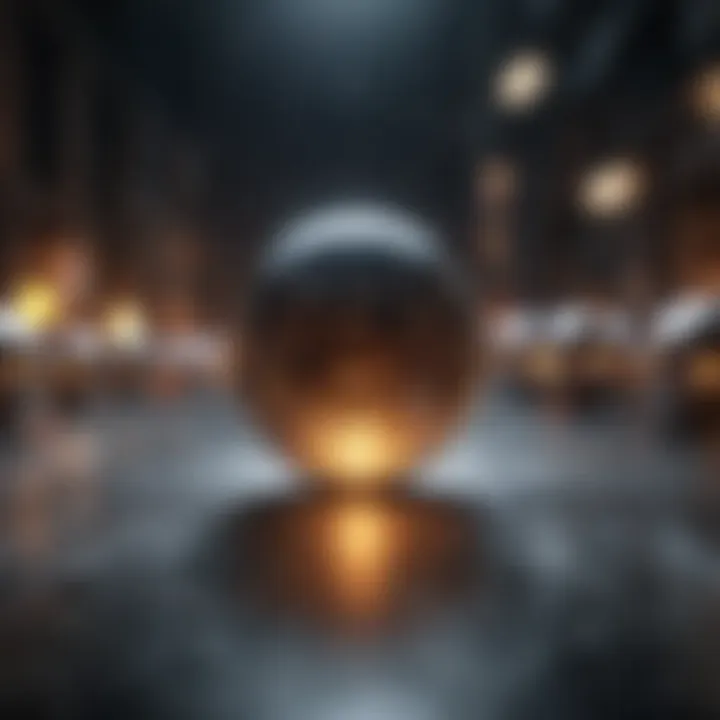
Moreover, in biomedical applications, nanoparticles are employed as imaging agents. By optimizing quantum yield, researchers are able to develop better diagnostic tools. Higher quantum yields improve the brightness of the signals emitted, leading to clearer imaging results, which is vital for early disease detection.
In summary, the intertwining of quantum yield and nanotechnology not only enhances current technologies but also propels innovation forward. Understanding these connections allows for targeted research and development efforts, ultimately paving the way for breakthroughs in multiple sectors.
Challenges in Quantum Yield Studies
Understanding the challenges in quantum yield studies is essential for advancing both theoretical and experimental research. A comprehensive grasp of these challenges enables scientists to refine techniques and develop more reliable results. The significance of addressing these challenges remains at the forefront of ongoing studies in the field. Improving measurement consistency and understanding the limitations of current techniques can enhance the utility of quantum yield in various applications.
Variability in Measurements
Variability in quantum yield measurements is a common issue that researchers face. Many factors can contribute to discrepancies in results, including instrument calibration, sample purity, and environmental conditions. For instance, slight differences in light source intensity can lead to significant deviations in quantum yield calculations. Additionally, the method of sample preparation can impact the outcome, especially in complex biological systems or in nanomaterials.
Researchers must recognize that quantum yield is not a fixed value. Rather, it can fluctuate based on experimental conditions. Thus, adequately reporting all variables is essential for reproducibility. To tackle this variability, standardization of measurement protocols is critical.
To summarize, here are some points to consider regarding variability in measurements:
- Inconsistencies in instrumentation: Different devices may yield varying results.
- Sample preparation techniques: Variations may lead to alterations in quantum yield.
- Environmental factors: Temperature and humidity affect measurements.
Limitations of Current Techniques
Current techniques for measuring quantum yield also face their own set of limitations. While many methods exist, each has unique constraints that researchers must navigate. For example, direct measurement methods can be very accurate but often require complex setups that may not be feasible in all laboratories. Indirect methods, while simpler, may introduce additional assumptions that can skew results. This trade-off between accuracy and convenience presents a significant hurdle.
Some notable limitations include:
- Sensitivity to changes: Many techniques need finely controlled conditions to produce valid data.
- Calibration challenges: Instruments may require frequent calibration leading to delays.
- Complexity of biological systems: Measuring quantum yield in live cells or organisms adds layers of difficulty due to multiple variables.
Future Directions
Furthermore, the understanding of quantum yield is likely to evolve beyond its current limitations. Innovations in measuring techniques are not just enhancing accuracy but also enable more sensitive detection methods. This will assist in obtaining data under various environmental and chemical conditions.
Interdisciplinary collaboration plays a crucial role in this evolution. Scientists from third domains can share insights, fostering advancements that solely focused research may miss. For example, combining knowledge from physics and biology might lead to new strategies for enhancing energy efficiency in photosynthesis.
Interdisciplinary Approaches
Interdisciplinary approaches address quantum yield not as an isolated concept but as one impacting many fields. A prime example is the merging of photochemistry and nanotechnology. Understanding how quantum yield functions at the nanoscale could lead to breakthroughs in solar energy conversion. In a similar vein, merging quantum mechanics with biological principles could unveil deeper layers of cellular processes related to energy transfer and biophotonics.
Recent studies in materials science propose new methods of synthesizing compounds that optimize quantum yield in varied environments. These collaborations expand the potential applicability of quantum yield beyond traditional boundaries, allowing researchers to explore uncharted territories in science.
"Interdisciplinary collaboration can yield insights that push the boundaries of our understanding in quantum yield."
Potential for Innovation
The future of quantum yield holds immense potential for innovation. One can envision novel materials engineered at the molecular level to enhance quantum yield in applications ranging from drug delivery systems to photovoltaic devices. New methods will likely emerge, permitting fine-tuning of quantum yield to achieve desired effects in technology and medicine.
Moreover, advancements in fluorescence techniques stemming from a deeper knowledge of quantum yield can revolutionize medical imaging. Enhanced imaging technologies could result from this understanding, enabling earlier diagnosis and treatment of diseases.
In summary, as the scientific community embraces the future of quantum yield, there will be a marked shift in how we approach and apply this integral concept across various disciplines. Continuous research efforts and collaboration will amplify the potential that lies within this fundamental aspect of photonic processes.
Culmination
As we wrap up our exploration of quantum yield, it is essential to recognize its foundational role in various scientific disciplines. Quantum yield, which measures the efficiency of photon-to-chemical transformation, is not just a theoretical concept. Rather, it has practical implications that can influence real-world applications and innovations across chemistry, physics, and biology.
"The study of quantum yield has far-reaching implications on both macro and micro scales, influencing everything from energy efficiency in plants to advancements in nanotechnology."
Furthermore, we have examined how environmental and chemical conditions can alter yield perceptions, underlining why specific methodologies must be chosen carefully. The interplay between instrumentation and methodology in obtaining accurate measurements is a significant takeaway that professionals should consider moving forward. In short, the insights provided here pave the way for further interrogation of quantum yield in various applications.
Summary of Key Points
- Definition and Importance: Quantum yield quantifies how efficiently absorbed energy is converted into a desired output, directly influencing many scientific disciplines.
- Measurement Techniques: Different methodologies, such as direct and indirect methods, as well as the instrumentation involved, play a critical role in obtaining precise quantum yield values.
- Influencing Factors: Several elements, including environmental and chemical conditions, can impact quantum yield, emphasizing the need for tailored approaches in experiments.
- Applications: Quantum yield is crucial for understanding processes in photosynthesis, photochemistry, and fluorescence, with significant implications in both practical and theoretical realms.
- Future Directions: Ongoing research is necessary to address the challenges in quantum yield studies and to harness its potential in emerging technologies, such as nanotechnology.
Importance of Continued Research
Continued research into quantum yield is paramount for several reasons. First, as scientific inquiry progresses, new insights and technologies emerge. These advancements can lead to better measurement techniques, enhancing our understanding of quantum yield further. New applications in energy transfer, phototherapy, and environmental science can be informed by deeper analyses of quantum yield. As interdisciplinary collaboration increases, bringing together chemists, physicists, biologists, and engineers, the potential for innovation also rises.
Moreover, understanding the nuances of quantum yield can inform sustainability and efficiency strategies in energy systems and technologies. By fine-tuning how we measure and interpret quantum yield, researchers can significantly contribute to advancements in renewable energy, providing a more sustainable future.
In summary, the journey into quantum yield does not end with this article. Rather, it marks the beginning of an exploration that calls for ongoing inquiry, collaboration, and innovation within the scientific community.