Converting Protein Sequences to DNA: Molecular Insights
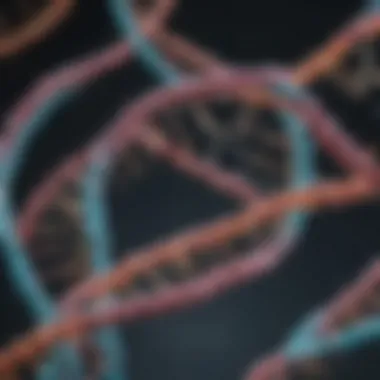
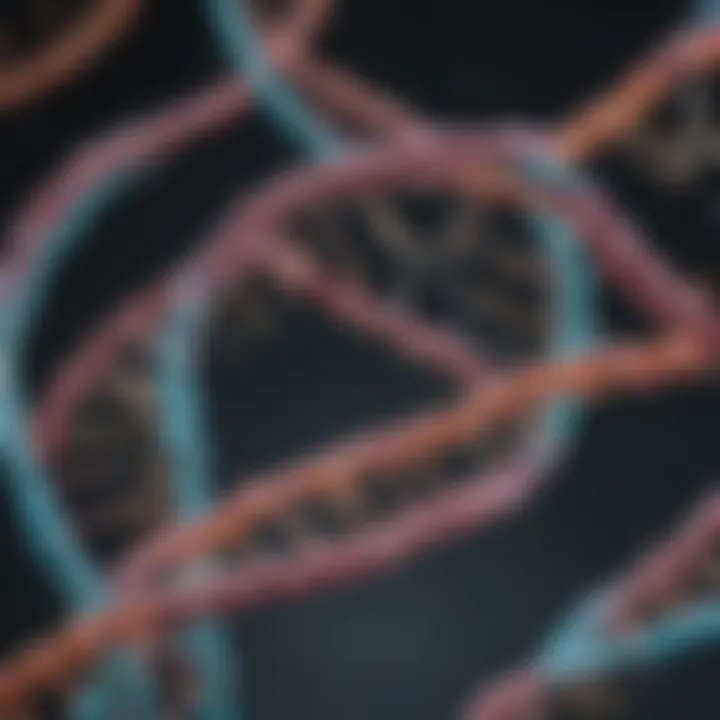
Intro
In the realm of molecular biology, the intricate relationship between proteins and DNA plays a critical role in the understanding of biological systems. Proteins, which are essential for almost every function in living organisms, are composed of amino acids. These proteins are synthesized based on the information encoded in the DNA sequence. But what if we could reverse this process? What if we could derive DNA sequences directly from proteins? This fascinating area of study bridges the gap between two fundamental aspects of biology, highlighting methodologies that have significant implications for genetic engineering and synthetic biology.
This exploration not only unravels the molecular mechanisms that facilitate the conversion of protein sequences into DNA but also provides insights into the broader context of biotechnology. Utilizing techniques such as reverse translation and genetic algorithms, researchers are able to create corresponding DNA sequences from given protein structures. This synergy is not merely a theoretical exercise; it has practical ramifications in areas such as gene therapy, vaccine development, and the creation of novel biomolecules.
The following sections will delve into the critical research highlights, summarize original studies in this domain, and dissect the methodologies that contribute to our understanding of this conversion process.
Foreword to Protein and DNA
The relationship between protein and DNA is central to molecular biology. Understanding this connection is essential as it lays the groundwork for numerous scientific advancements. The conversion of protein sequences into DNA not only enhances our comprehension of genetic mechanisms but also has applications in diverse fields such as biotechnology, medicine, and environmental science.
Proteins are the building blocks of life, serving various functions within organisms. They are crucial for catalyzing biochemical reactions, providing structural support, and carrying out cellular communication. A clear understanding of proteins is needed to appreciate their conversion into DNA sequences.
Conversely, DNA is the molecular repository of genetic information. Its structure and function are foundational for understanding biological systems. The process of translating proteins back into DNA allows researchers to manipulate genetic material more effectively, paving the way for innovative solutions in research and development.
Through this article, we will explore the mechanisms underlying the conversion from protein sequences to DNA, elucidating the methodologies involved and their implications for scientific pursuits.
Defining Proteins
Proteins are complex molecules composed of long chains of amino acids. The sequence of amino acids determines a protein's unique structure and functional properties. There are 20 different amino acids that can combine in various sequences to produce a linear chain, which then folds into a specific three-dimensional shape. The folded protein can adopt various forms and perform specific functions in an organism.
Proteins exist in several categories based on their roles:
- Enzymes: Catalysts that facilitate biochemical reactions.
- Structural Proteins: Provide support and shape to cells and organisms.
- Transport Proteins: Carry molecules across cellular membranes.
- Antibodies: Proteins that immune system uses to identify and neutralize pathogens.
Understanding protein structure and function is critical to grasping how these molecules can be synthesized from their corresponding genetic codes, which leads us to examine the nature of DNA.
Understanding DNA
DNA, or deoxyribonucleic acid, is the molecule that encodes genetic information. It consists of two strands forming a double helix structure, with each strand composed of nucleotides. Each nucleotide contains a sugar, a phosphate group, and a nitrogenous base. There are four types of nitrogenous bases in DNA: adenine, thymine, cytosine, and guanine. The sequence of these bases constitutes the genetic code that instructs cells to produce proteins.
The organization of DNA occurs in chromosomes within the nucleus of eukaryotic cells. The human genome, for example, contains over 3 billion base pairs that encode approximately 20,000-25,000 genes.
The ability to parse the relationship between DNA and protein sequences is crucial for many applications in molecular biology. Various methods, such as PCR and sequencing, can analyze DNA to identify genetic disorders or understand evolutionary relationships. This foundational knowledge will be vital as we explore the mechanisms of converting protein sequences into DNA in subsequent sections.
The Central Dogma of Molecular Biology
The Central Dogma of Molecular Biology outlines the flow of genetic information from DNA to RNA to proteins. Understanding this foundational framework is essential as it provides insights into how proteins, which serve as functional molecules in living organisms, are synthesized from DNA. This article primarily concerns how the conversion of protein sequences back into DNA sequences can have significant implications in synthetic biology and genetic engineering.
The importance of the Central Dogma lies in its delineation of the processes that govern biological functions at the molecular level. The principles articulated by this dogma are fundamental for biologists, educators, and researchers who strive to unravel the complexities of genetic expression and manipulation.
DNA to RNA Transcription
Transcription is the first critical step in the Central Dogma. During this process, the DNA sequence of a gene is transcribed into messenger RNA (mRNA). This step is essential because it serves as a bridge between the genetic material in DNA and the synthesis of proteins. RNA polymerase is the key enzyme involved, unwinding the DNA helix and synthesizing a strand of RNA complementary to the DNA template.
This mRNA strand carries the genetic code necessary for protein synthesis. Understanding transcription facilitates the decoding of specific proteins from their sequence, which is necessary for subsequent applications in biotechnology and gene therapy.
RNA to Protein Translation
Following transcription, the next step is translation. During translation, the mRNA is translated into a specific sequence of amino acids, forming a protein. Ribosomes, along with transfer RNA (tRNA), play an essential role in this process. Each tRNA has an anticodon that pairs with a codon on the mRNA strand, ensuring the correct amino acids are incorporated.
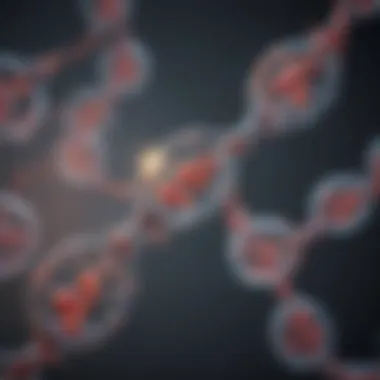
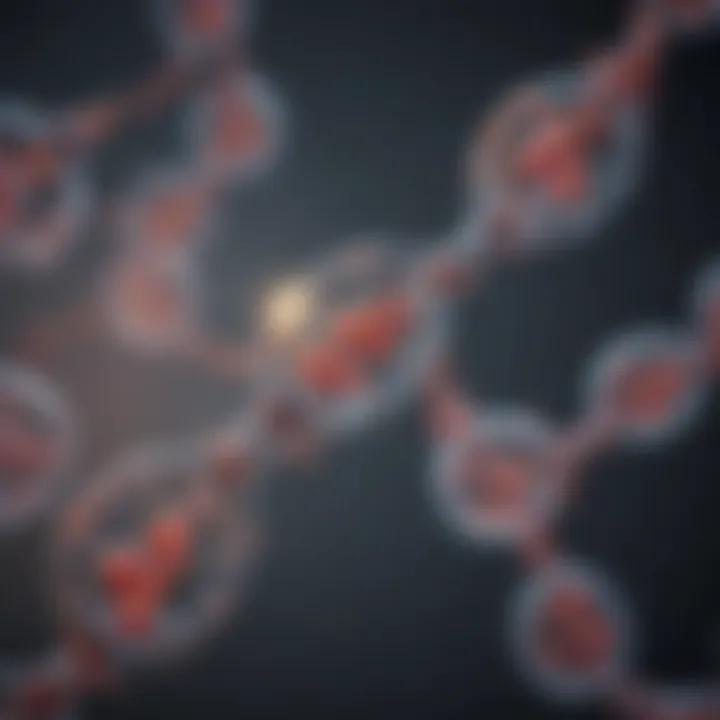
The translation process is significant as it results in the formation of proteins that perform a variety of functions within cells, including catalyzing biochemical reactions, providing structural support, and regulating biological processes. Understanding translation is vital when considering how to convert protein information back into DNA sequences.
Reverse Processes and Their Implications
Reverse processes, such as reverse transcription, have important implications in molecular biology. While the Central Dogma describes a one-way flow of information, reverse transcription allows RNA to be converted back into DNA. This is exemplified in retroviruses like HIV, which utilize reverse transcriptase to transcribe their RNA genome into DNA that integrates into the host genome.
The understanding of these reverse processes is crucial in genetic engineering and synthetic biology. They present opportunities for manipulating genetic sequences for research, therapeutic interventions, and biotechnological applications.
"The Central Dogma not only describes the flow of genetic information but also highlights the dynamic nature of molecular processes that underpin cellular function."
By comprehending the central dogma, including the reverse processes, scientists and researchers gain a profound understanding of how genetic information can be manipulated, providing further insight into the conversion of proteins back into their corresponding DNA sequences. This knowledge is indispensable for future advancements in the field of synthetic biology.
From Protein Sequences to Genetic Code
The relationship between protein sequences and the underlying genetic code is foundational in molecular biology. Understanding how proteins are translated back into DNA offers insights into cellular functions and genetic expression. This process allows researchers to manipulate biological systems at a fundamental level, enhancing our ability to engineer new proteins, understand diseases, and create innovative biotechnological applications. The topic of this section highlights how each protein, made of long chains of amino acids, corresponds to specific sequences of nucleotides in DNA.
Amino Acids and Codons
Amino acids serve as the building blocks of proteins. There are twenty standard amino acids, and they combine in various sequences to form different proteins. Each amino acid corresponds to a triplet of nucleotides known as a codon in the genetic code. The significance of codons lies in their ability to dictate which amino acids are assembled into proteins during the process of translation.
To elaborate further:
- Codon Usage: Each codon can specify only one amino acid but multiple codons can code for a single amino acid. For example, leucine is represented by six different codons: CUU, CUC, CUA, CUG, UUA, and UUG.
- Universal Nature: The genetic code is nearly universal across all known living organisms, making it easier to study and engineer genes across species.
Determining the sequences of amino acids in a protein enables scientists to decode the corresponding codons from the DNA. This decoding process is critical because it lays the foundation for genetic engineering, allowing scientists to synthesize new proteins that could one day advance medicine and other fields.
Decoding Protein to DNA
The transition from protein sequences back to DNA sequences is non-trivial but essential for scientific advancements. This decoding process involves determining the precise nucleotide sequences that would produce a desired protein. Here, each amino acid in a sequence requires the identification of one or more specific codons that could represent it. Expertise in bioinformatics aids scientists in reconstructing the DNA code efficiently.
- Identify the Protein Sequence: Start by analyzing the specific amino acid sequence of the protein in question.
- Map to Codons: Utilize known codon tables to find possible corresponding DNA sequences for each amino acid. This involves consideration of preferred codon usage in the target organism.
- Synthesize the DNA: Through chemical synthesis or cloning techniques, create the DNA sequence in a lab environment.
The process of converting a protein sequence back to DNA opens pathways for engineering novel proteins, with implications for fields ranging from biotechnology to medicine.
Understanding these conversions holds immense potential. It enables tailored gene creation which leads to better therapeutic strategies, improved agricultural practices, and innovative solutions to environmental problems. This ability to decode protein to DNA seamlessly underscores the intricate tapestry of genetic expression in living organisms.
Methods for Protein to DNA Conversion
The conversion of protein sequences into DNA is a complex but vital process in molecular biology. Understanding the methods used for this conversion can illuminate the potential benefits and implications in various fields such as biotechnology and synthetic biology. Precise conversion not only aids in studying proteins but also enhances genetic engineering practices. When we discuss methods for protein to DNA conversion, we focus on several key approaches that involve technological advancements and computational tools.
Synthetic Biology Techniques
Synthetic biology has emerged as a pivotal field that combines biology and engineering principles. One of the core techniques in this area is the use of genetic parts, which allow scientists to build new biological systems or modify existing ones.
- Gene Synthesis: This is a technique where scientists create DNA sequences from scratch, designing them to match the amino acid sequence of a given protein. This enables the creation of custom genes tailored for specific functions or studies.
- Assembly Methods: Techniques such as Gibson assembly or Golden Gate assembly enable the joining of multiple DNA fragments together into a complete gene sequence. This is particularly useful when converting longer protein sequences, as it allows for modular assembly of the corresponding DNA.
These methods enhance the precision of creating genetic constructs that can express desired proteins effectively. They also facilitate exploring complex pathways in organisms, which could lead to innovative solutions in medicine and environmental science.
Bioinformatics Approaches
Bioinformatics plays a critical role in analyzing biological data and converting protein sequences to DNA. It involves computational tools that can predict the genetic code corresponding to a protein.
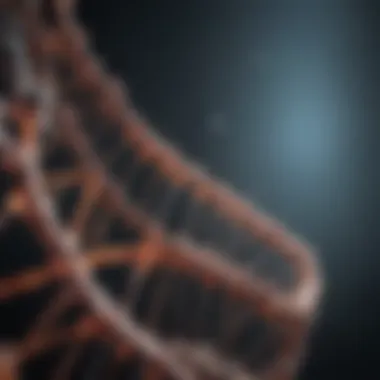
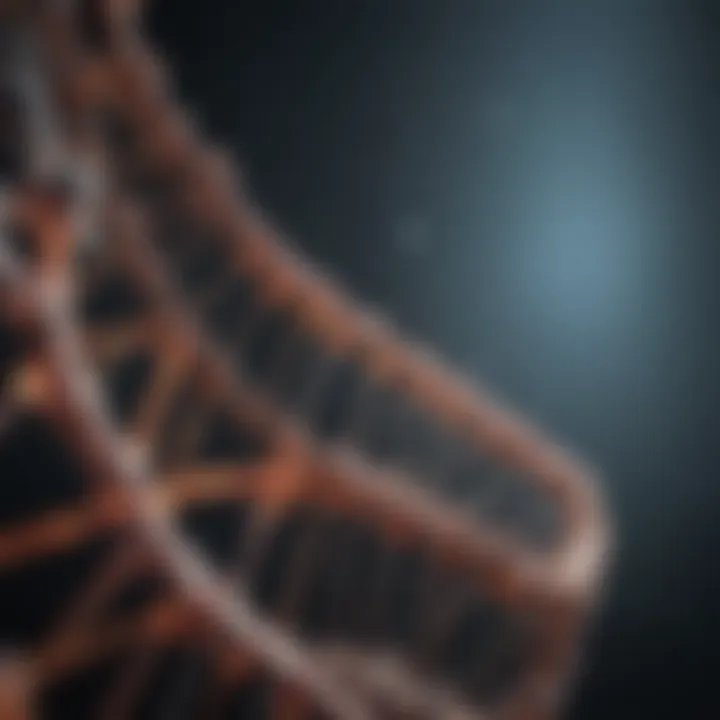
- Sequence Alignment: Algorithms like BLAST (Basic Local Alignment Search Tool) allow researchers to compare protein sequences with known databases to identify the best theoretical DNA sequences.
- Codon Optimization: Different organisms prefer different codons for the same amino acids. Bioinformatics tools can optimize these codons to ensure high expression levels in target organisms, maximizing protein yield during experimentation.
These bioinformatics approaches not only streamline the conversion process but also enhance accuracy by leveraging vast datasets and sophisticated algorithms.
Computational Methods for Sequence Prediction
Advancements in computational methods significantly contribute to the efficiency of protein to DNA conversion. These methods utilize machine learning and statistical models to predict the most likely DNA sequence that would encode a specific protein.
- Machine Learning Models: Algorithms can be trained on large datasets of known protein sequences and their corresponding DNA. By learning patterns, these models can predict sequences more accurately.
- Statistical Models: Tools such as Markov Chain models or Hidden Markov Models can infer the probable DNA structure needed given the protein characteristics.
These computational methods not only improve the speed of the conversion process but also aid in the design of new proteins through in silico studies.
The ability to convert protein sequences to DNA effectively opens numerous avenues for research and application in molecular biology, ultimately leading to enhanced capabilities in genetic engineering and synthetic biology.
Applications of Protein to DNA Conversion
The conversion of protein sequences into DNA sequences plays a critical role in various fields of molecular biology and biotechnology. By understanding how to translate amino acid sequences into their corresponding nucleotide sequences, researchers can design and create new proteins, develop innovative therapies, and address environmental challenges. This section highlights the specific applications of protein to DNA conversion in multiple domains.
Biotechnology and Synthetic Gene Creation
In biotechnology, the ability to convert protein sequences into DNA is essential for synthetic gene creation. This process allows scientists to replicate natural proteins and engineer synthetic versions with improved properties. By designing genes that encode specific proteins, researchers can produce them in large quantities using microbial or cellular systems, a process known as recombinant DNA technology.
The applications of synthetic genes in biotechnology range from the production of therapeutic proteins, such as insulin, to the development of biofuels. Companies like Amgen and Genentech are leading examples of how synthesized proteins can revolutionize medicine by providing targeted treatments for complex diseases. Furthermore, synthetic genes enable the exploration of protein functionalities, this opens new avenues in enzyme design and modification for industrial processes.
Genetic Engineering in Medicine
Genetic engineering is another domain where the conversion of protein to DNA is of utmost importance. This approach allows for the manipulation of genetic material to create genetic therapies for various diseases. For instance, by substituting or adding specific genes responsible for protein deficiencies, researchers can develop treatments for genetic disorders.
Examples include:
- Gene therapy for hemophilia: Patients can receive a functional copy of the gene that codes for clotting factors, ensuring proper blood coagulation.
- CAR T-cell therapy: Patients' T-cells are genetically modified to express synthetic receptors that enhance their ability to target cancer cells.
These advancements demonstrate the potential to alter the genetic landscape of human cells, providing solutions to previously untreatable conditions. However, ethical concerns and regulatory frameworks need to be addressed as genetic manipulation raises significant societal questions.
Environmental Bioremediation Strategies
Environmental applications of protein to DNA conversion focus on remediating contaminated environments. Engineers can create microbes or plants that express specific proteins capable of breaking down pollutants. This potential aligns with growing environmental concerns due to industrial activities and waste.
Examples of these strategies include:
- Hydrocarbon-degrading bacteria: Bacteria can be engineered to express enzymes that degrade oil spills, leading to effective clean-up efforts.
- Phytoremediation: Plants can be genetically modified to absorb heavy metals from soil, thereby detoxifying contaminated sites.
The development of such bioengineered organisms not only helps to restore ecosystems but also contributes to sustainable practices in industries. As society moves towards greener technologies, the conversion of protein sequences into DNA can play a pivotal role in addressing ecological challenges.
"By transforming our understanding of proteins and their corresponding sequences, we lay the groundwork for innovations across medicine, environment, and technology."
Through these applications, the importance of protein to DNA conversion cannot be overstated. It facilitates breakthroughs in medicine, supports sustainable practices, and keeps driving forward the fields of biotechnology and environmental science.
The Ethical Considerations
The exploration of converting protein sequences into DNA raises numerous ethical considerations that must be diligently assessed. These considerations span the realms of biotechnology, genetic engineering, and the broader implications of manipulating life at the molecular level. In discussing these ethical facets, it's essential to frame concerns around the potential benefits and drawbacks tied to genetic manipulation.
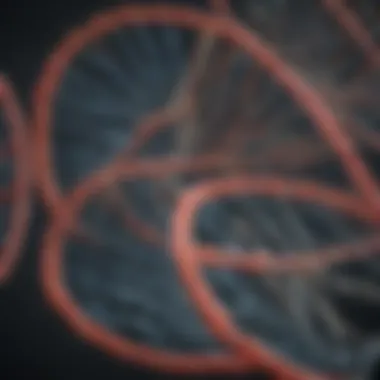
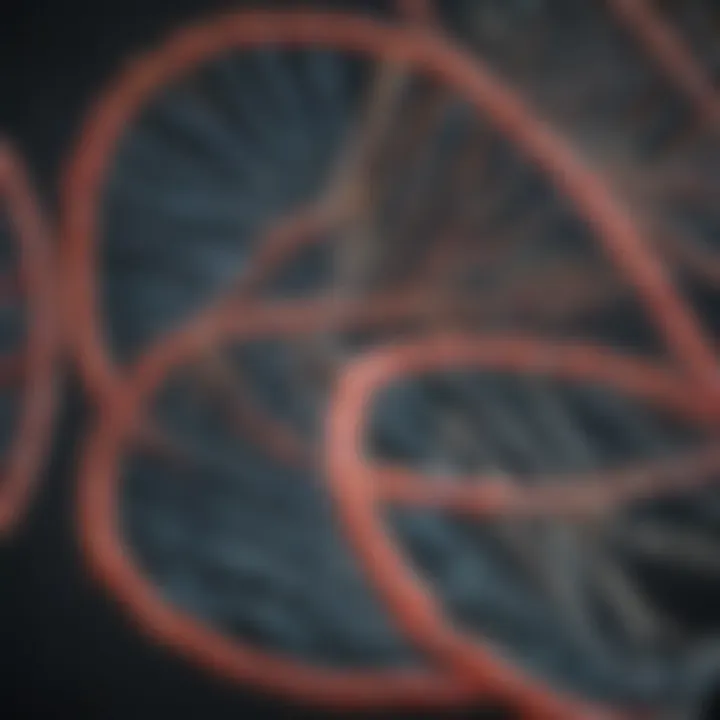
Concerns in Genetic Manipulation
As scientific advances enable the manipulation of genetic material, various concerns emerge. Firstly, there is apprehension regarding biosecurity. The possibility of creating harmful organisms or bio-weapons cannot be overlooked. The prospect of designing pathogens with increased virulence could pose significant threats to public health.
Another concern is related to biodiversity. The introduction of genetically modified organisms (GMOs) into the environment could disrupt existing ecosystems. These alterations may lead to unintended consequences, like the extinction of native species or the emergence of superweeds resistant to herbicides. The risk of altering natural selection processes raises questions about the long-term viability of ecosystems.
Moreover, ethical issues regarding consent and the right to modify organisms come into play. In the context of medicine, should patients and families have a say in the genetic modifications being made? Furthermore, the implications of heritable genetic changes mean that future generations are affected without their consent, which generates an ongoing ethical debate.
Regulatory Frameworks
Addressing these ethical concerns necessitates established regulatory frameworks that govern genetic manipulation practices. Regulations need to encompass safety assessments, risk analysis, and ongoing monitoring of genetically modified organisms. Many countries have varying regulations, creating a patchwork of rules that can complicate compliance for researchers and companies.
Some key points in current regulatory frameworks include:
- Pre-market Assessment: Organizations such as the U.S. Food and Drug Administration (FDA) and the European Food Safety Authority (EFSA) impose strict guidelines for evaluating GMOs before entering the market.
- Environmental Impact Assessments: These are essential to assess the potential effects of releasing modified organisms into the environment.
- Public Engagement: Regulatory bodies increasingly recognize the need for public input in decision-making related to genetic modifications.
p>As technology advances, there is an ongoing need for adaptive regulations that can keep pace with the rapid developments in synthetic biology.p>
"The regulation of biotechnology must not only involve scientists but also ethical scholars, environmentalists, and the affected communities."
Future Directions in the Field
As the study of converting protein sequences into DNA continues to evolve, several future directions have emerged. These advancements are crucial in understanding and expanding the potential applications of molecular biology. The exploration of new techniques holds the promise to enhance efficiency, accuracy, and scope of gene synthesis. Furthermore, addressing the complexities of these methods will facilitate genetic advancements, improving the potential of biotechnology.
Advancements in Gene Synthesis Techniques
Gene synthesis techniques have seen remarkable progress over the years. The most significant developments include:
- Error Correction: Innovations in error correction methods enhance the fidelity of synthesized gene sequences, reducing the likelihood of mutations.
- Scalability: Recent approaches allow for large-scale synthesis, making the production of multiple gene sequences more manageable and cost-effective.
- High-Throughput Technologies: These methods accelerate the synthesis process, allowing researchers to generate extensive libraries of genes quickly.
The ongoing improvements in these techniques will undoubtedly advance our ability to manipulate genetic material efficiently. The focus on increasing precision in gene synthesis will enable scientists to create more reliable synthetic genes, which is essential in developing therapeutic avenues and biotechnological solutions.
Impact of Artificial Intelligence on Synthetic Biology
Artificial intelligence (AI) is revolutionizing the field of synthetic biology. By leveraging AI technology, researchers can drive efficiencies in gene design and analysis. Some key impacts include:
- Predictive Modeling: AI tools help predict the outcomes of genetic modifications, enabling scientists to refine experiments before actual implementation.
- Data Analysis: Machine learning algorithms can analyze vast datasets generated by genetic experiments, uncovering patterns that may be overlooked by traditional methods.
- Optimizing Pathways: AI can help in designing genetic circuits that optimally express proteins, increasing the chance of desired outcomes in synthetic biology applications.
The integration of AI into the field will likely lead to significant breakthroughs in gene synthesis and protein function prediction. With tools that can adjust strategies in real-time based on experimental data, researchers may achieve faster and more robust results in synthetic biology.
Closure
The conclusion of this article addresses the vital role the conversion of protein sequences into DNA plays in the broader context of molecular biology. This topic is not just a matter of scientific curiosity; it bears significant implications for various fields, including biotechnology, medicine, and environmental science. The ability to move from protein manifestations back to DNA forms provides a deeper understanding of genetic coding and expression. This cyclic nature of biology underscores the interdependence between proteins and nucleic acids, facilitating a multi-faceted approach in research and application.
Recapitulating Key Insights
In summary, a few key insights emerge from the exploration of protein to DNA conversion:
- The central dogma establishes a foundational framework for understanding genetic information flow.
- Amino acids, the building blocks of proteins, correspond to specific codons in DNA, making translation possible.
- Various methods, such as synthetic biology techniques and bioinformatics, are available to achieve successful conversions.
- Applications extend into practical realms, enabling advancements in synthetic gene creation and environmental bioremediation strategies.
These insights demonstrate that the conversion process is a critical bridge in molecular biology that aligns with both theoretical and practical aspects of scientific inquiry. Understanding this process connects researchers to the molecular tapestries that constitute life itself.
The Significance of Continued Research
Continued academic and practical investigation into the protein to DNA conversion process is increasingly important. The potential benefits are far-reaching. For instance, advancements in gene synthesis techniques present opportunities for tailored medical therapies, enhanced agricultural resistance, and improved bioremediation practices.
Research in this area contributes to a robust understanding of how variations in protein coding can influence organismal behavior and health. Furthermore, integrating artificial intelligence into synthetic biology holds the promise of streamlining research, improving accuracy in sequence predictions, and potentially revolutionizing molecular biology as a discipline.
Emphasis on interdisciplinary collaboration will be essential to foster innovation. By combining insights from genetics, molecular biology, computational methods, and ethics, the next era of discoveries can be more strategic and impactful. Overall, the significance of continued exploration in converting protein sequences into DNA cannot be overstated—this work may unlock future applications that fundamentally alter our interaction with biology.