Exploring Biocompatible Polymers: Innovations & Applications
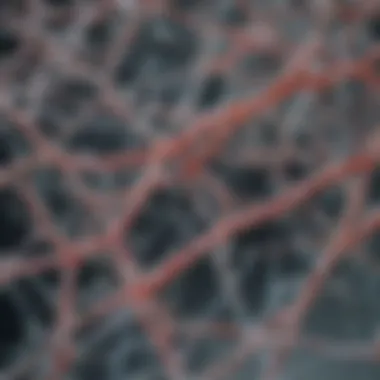
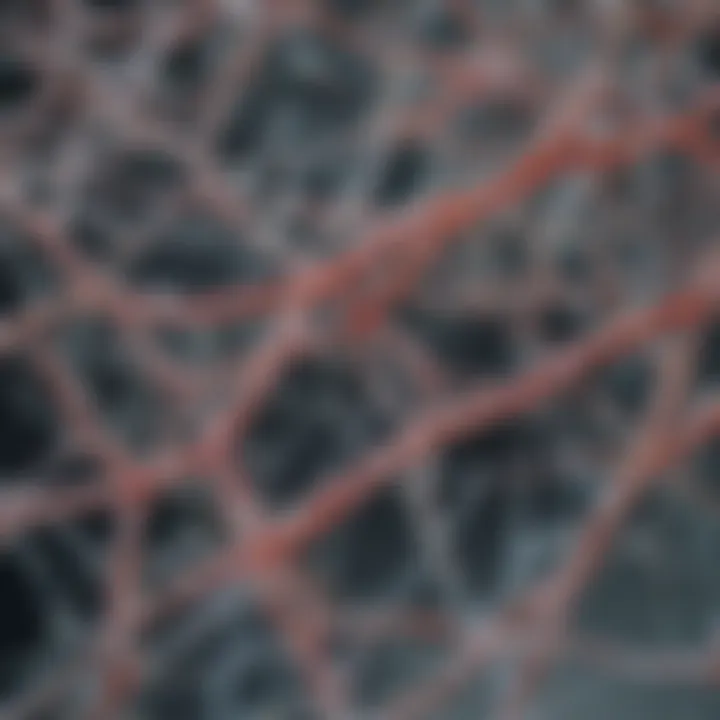
Intro
Biocompatible polymers play a crucial role in the intersection of medicine, industry, and the environment. Their unique properties allow them to interact with biological systems without eliciting an immune response. This has led to widespread applications, particularly in medical fields such as drug delivery, tissue engineering, and implants. As the demand for innovative materials increases, understanding the nuances of biocompatibility and how these polymers function becomes essential.
The synthesis of biocompatible polymers can be approached through various methods, revealing a spectrum ranging from natural to synthetic options. This versatility has facilitated rapid progress in research and application, leading to numerous innovations. Despite these advancements, challenges remain regarding regulatory approval and practical implementation, which the research community continues to address.
The following sections will provide a detailed overview of the key findings in this area. Each aspect is significant in understanding the evolving landscape of biocompatible polymers and their implications for future research and technologies.
Research Highlights
Overview of Key Findings
Biocompatible polymers exhibit essential properties such as flexibility, strength, and compatibility with human tissues. The research highlights include:
- Various types of biocompatible polymers, including polyethylene glycol, polylactic acid, and collagen.
- Application of these polymers in drug delivery systems that enhance the bioavailability and control the release of therapeutic agents.
- The role of these materials in tissue engineering, aiding in the regeneration of damaged tissues through scaffolding applications.
These findings underline the critical necessity for continuous research and the development of advanced materials that can cater to specific health needs.
Significance of the Research
Understanding biocompatible polymers is not only vital for developing medical devices but also for their influence on environmental sustainability. For instance, some biodegradable polymers help minimize medical waste, illustrating the intersection of healthcare and ecological awareness.
"Advancements in biocompatible polymers reflect a journey toward more sophisticated and effective medical solutions."
Their significance extends beyond the laboratory and manufacturers, impacting patients' lives and global health conditions. As researchers and industries harness these materials, they create a pathway for innovations that align with modern challenges.
Original Research Articles
These findings are often published in peer-reviewed journals, which provide substantial contributions to the field.
Summary of the Articles
Research articles focus on synthesizing novel biocompatible materials, evaluating their effectiveness in real-world applications, and exploring regulatory pathways. Each study adds to the knowledge base, informing both practical applications and future research directions.
Author Contributions
The collaborative nature of this research field requires that various experts contribute via interdisciplinary approaches. Together, scientists from materials science, biology, and engineering contribute unique insights, which can lead to groundbreaking innovations in biocompatibility and polymers.
Understanding Biocompatible Polymers
In the realm of modern science, biocompatible polymers hold a critical place, particularly in the fields of medicine and environmental protection. Their relevance is underscored by a growing demand for materials that can effectively interact with biological systems without causing harm. Understanding biocompatible polymers is essential for professionals, educators, and researchers who are invested in innovative applications.
Biocompatibility refers to the ability of a material to perform with an appropriate host response in a specific application. This means that biocompatible polymers must not only be safe for the body but should also function effectively in their role, whether that be supporting drug delivery, serving as scaffolds in tissue engineering, or contributing to biodegradable solutions in environmental contexts.
With their significant versatility, these polymers create pathways for advancements in healthcare and sustainability. They can be tailored chemically and physiologically to suit diverse applications. Therefore, it is critical to explore their definitions, classifications, and functional characteristics.
Definition of Biocompatibility
Biocompatibility is a term that encapsulates the compatibility of a foreign material with the living body. It implies that when a biocompatible polymer interacts with biological tissues, it does so without adverse effects. This compatibility can stem from various factors, including chemical structure, mechanical properties, and degradation behavior. A key part of understanding biocompatibility is recognizing the importance of specific interactions at the molecular level, which can influence the performance of these polymers in medical and environmental applications.
Types of Biocompatible Polymers
Biocompatible polymers can be broadly categorized into two distinct groups: natural polymers and synthetic polymers. Each category presents unique properties and advantages that make them suitable for various applications.
Natural Polymers
Natural polymers, derived from biological sources, have inherent advantages that include biocompatibility and biodegradability. Some common examples include collagen, chitosan, and hyaluronic acid. One of the key characteristics of natural polymers is their ability to promote cellular functions, such as adhesion and proliferation, which is particularly beneficial in tissue engineering.
However, natural polymers can be limited by variability in source material, potential allergenicity, and variable mechanical properties. Despite these challenges, their inherent properties and biological versatility make them a popular choice in the development of medical devices and drug delivery systems.
Synthetic Polymers
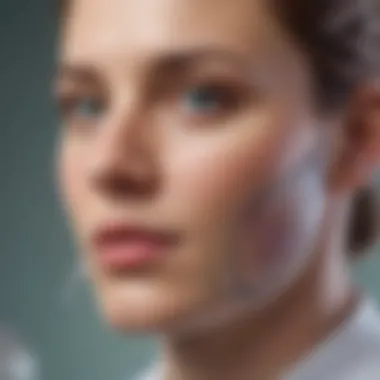
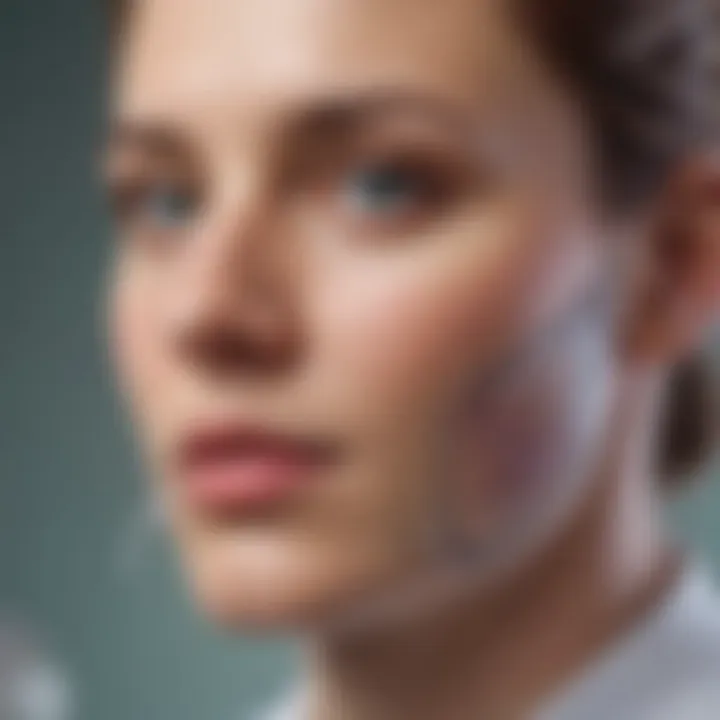
In contrast, synthetic polymers are designed and produced through chemical processes, allowing for a higher degree of control over their properties. Polymers such as polyethylene glycol and polylactic acid are common examples. The key characteristic of synthetic polymers lies in their configurable mechanical and chemical properties, which can be tuned to meet specific requirements.
The unique feature of synthetic polymers is their ability to be engineered for desired degradation rates and mechanical strength. They tend to offer consistent performance and can be mass-produced, making them an economical option. However, their biocompatibility is not always guaranteed, as some synthetic materials can elicit unwanted immune responses.
Through careful selection and thorough understanding of both natural and synthetic biocompatible polymers, researchers can drive innovations in multiple applications, from health care to environmental sustainability. This exploration of biocompatible polymers underscores the balance of benefits and limitations inherent in the field, shaping our understanding of how materials can best serve human needs.
"The realms of biocompatibility pave the way for future advancements in both medicine and environmental science, showcasing a blend of innovation and necessity."
Understanding these distinctions enriches the conversation on biocompatible polymers, allowing for better design, application, and research efforts moving forward.
Properties of Biocompatible Polymers
The properties of biocompatible polymers are crucial for understanding their role in various applications. These polymers must exhibit a set of characteristics to ensure they function properly and safely, particularly in medical and environmental uses. The importance of these properties lies in their direct impact on the effectiveness and safety of the materials utilized in real-world applications.
Mechanical Properties
Mechanical properties refer to a polymer's ability to withstand forces without breaking. This includes tensile strength, elasticity, and toughness. For any polymer intended for medical implants or scaffolds in tissue engineering, maintaining structural integrity under physiological conditions is vital.
- Tensile strength: This indicates how much force a material can endure when pulled or stretched. High tensile strength is essential for implants that must support mechanical loads in the body.
- Elasticity: This property describes the ability of a polymer to stretch and return to its original shape. In medical devices, elasticity can enhance comfort and functionality, especially in applications involving movement.
- Toughness: A tough polymer can absorb significant energy before cracking or failing. It is beneficial in devices that face sudden stresses, such as orthopedic implants.
Understanding these mechanical properties allows researchers and manufacturers to select the right materials for specific applications, ultimately improving both performance and patient outcomes.
Chemical Properties
Chemical properties of biocompatible polymers are equally important. These properties help define how a polymer interacts with its environment, including bodily fluids and other materials. Important elements include chemical stability, degradation rate, and bioreactivity.
- Chemical stability: A stable polymer does not react undesirably with bodily fluids or other substances. This stability reduces the risk of adverse reactions or side effects in medical applications.
- Degradation rate: For certain applications, controlled degradation is necessary. For instance, in drug delivery systems, polymers must break down at a specific rate to release drugs effectively.
- Bioreactivity: This property describes how the polymer interacts with biological tissues. Ideally, a biocompatible polymer should elicit minimal inflammatory response while promoting healing processes.
In summary, chemical properties significantly influence how biocompatible polymers perform in their intended settings, affecting their safety and utility.
Biodegradability
Biodegradability is a critical factor for both environmental and medical applications. A biodegradable polymer breaks down into harmless components over time in specific conditions, which is especially important for reducing long-term waste and environmental pollution. In the medical field, biodegradability is advantageous for controlled drug release and temporary implants.
- Environmental impact: Biodegradable polymers contribute to sustainability by minimizing waste accumulation in landfills.
- Tissue regeneration: In tissue engineering, materials that can degrade and be replaced by natural tissue can promote better healing and integration.
Applications in Medicine
Biocompatible polymers have significantly influenced modern medicine through various applications. They provide essential functions that enhance patient care, promote healing, and improve medical outcomes. The versatility of these materials allows for their use in multiple domains, including drug delivery, tissue engineering, and implantable devices. As healthcare continues to evolve, the need for sophisticated solutions becomes more apparent, highlighting the importance of these polymers in addressing complex biomedical challenges. They offer specific benefits such as improved biocompatibility, tailored mechanical properties, and biodegradability, which are crucial in medical settings.
Drug Delivery Systems
In drug delivery systems, biocompatible polymers play a pivotal role in enhancing the therapeutic effectiveness of medications. They facilitate controlled release mechanisms, which ensure that drugs are delivered at a consistent rate over time. This trait is particularly beneficial for chronic diseases, where steady medication administration is crucial. The ability to personalize drug formulations using these polymers allows scientists to optimize treatment regimens tailored to individual patient needs. Significant types of drug delivery systems include liposomes and micelles, which utilize polymeric carriers to encapsulate and transport drugs to specific target sites in the body.
Tissue Engineering
Scaffolding Materials
Scaffolding materials are fundamental in tissue engineering. These polymers provide a physical structure to support cell growth and tissue regeneration. A key characteristic of scaffolding materials is their porous nature, which enables nutrient flow and waste removal, essential for cell survival. The wide range of available materials, including collagen and polylactic acid, have made them popular choices for researchers. Unique features, such as tunable degradation rates, allow scaffolds to be designed to match the natural healing rates of tissues, providing a synergistic environment that promotes natural healing processes. However, following successful healing, the challenge lies in ensuring the scaffold does not elicit adverse reactions or toxicity over time.
Cell Encapsulation
Cell encapsulation is another significant technique within tissue engineering. This process involves enclosing living cells within a semipermeable membrane, providing a protective environment. The key characteristic here is the selective permeability of the membranes that allows nutrients and waste to pass while keeping out harmful substances. This technique is beneficial for delivering cells that produce therapeutic agents or for transplanting insulin-producing cells for diabetes treatment. Unique features of cell encapsulation include immediate protection of implanted cells from the host's immune response, thus reducing rejection rates. Despite these advantages, there are challenges such as potential mass transfer limitations, which can affect cell viability.
Implantable Devices
Implantable devices utilize biocompatible polymers to ensure patient safety and comfort. Examples include stents, prosthetic devices, and drug-eluting implants. The significance of biocompatibility in these devices cannot be overstated, as poor integration can lead to complications such as inflammation or rejection by the body. These polymers are selected based on their mechanical properties and ability to withstand the body's environment for an extended period. Ongoing research focuses on improving the performance of these devices, aiming for better integration and functionality within the human body.
In summary, biocompatible polymers are at the forefront of medical innovations, bridging the gap between technology and healthcare. Their diverse applications help improve treatment outcomes and patient quality of life.
Environmental Applications
Environmental applications of biocompatible polymers are essential for addressing modern ecological challenges. These materials enable advances in sustainability and waste reduction, allowing for innovative solutions that can minimize environmental impact. Their unique properties make them suitable for various uses, particularly in fields like waste management and pollution control.
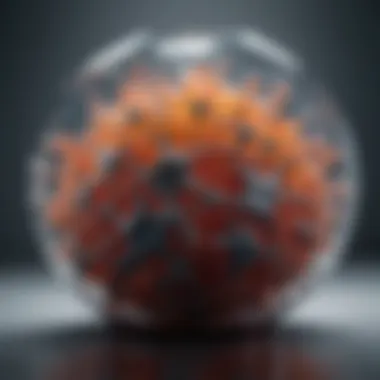
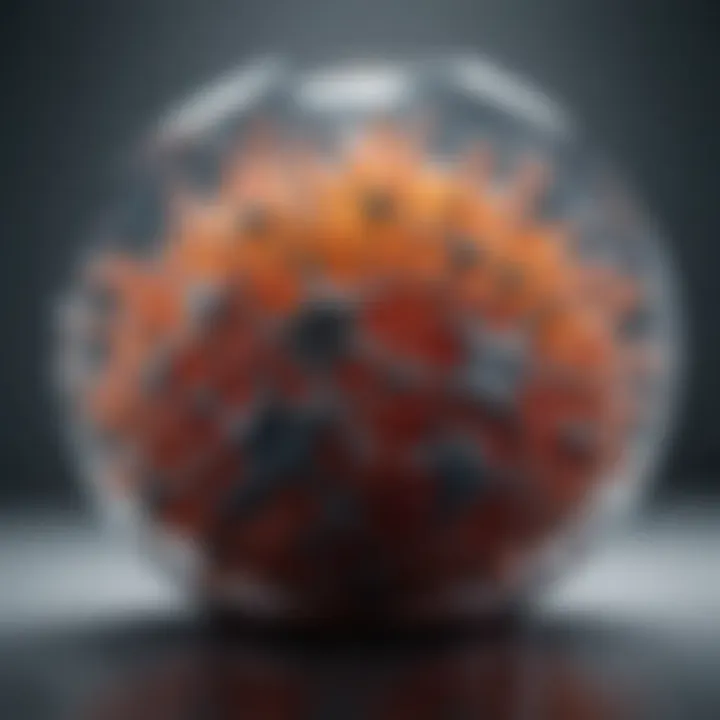
Biodegradable Plastics
Biodegradable plastics represent a significant advancement in polymer technology, offering a sustainable alternative to traditional plastics. Unlike conventional plastics that persist for hundreds of years in the environment, biodegradable plastics break down into natural substances over a relatively short period. This process occurs through microbial action, which is critical for reducing pollution.
The use of biodegradable plastics can greatly reduce the volume of waste sent to landfills. Their application spans multiple sectors, including packaging, agriculture, and consumer products. For example, biodegradable bags are now commonly used in grocery stores. Additionally, their implementation in agricultural films helps in soil improvement by decomposing and providing nutrients back to the soil.
Some examples of biodegradable polymers include:
- Polylactic Acid (PLA): Derived from renewable resources like corn starch, it is widely used in packaging and disposable tableware.
- Polyhydroxyalkanoates (PHAs): Produced through microbial fermentation, PHAs are suitable for medical applications as well as packaging.
"The shift toward biodegradable plastics not only addresses environmental concerns but also aligns with consumer demands for more eco-friendly products."
Water Purification Systems
Water purification is another critical area where biocompatible polymers have shown remarkable potential. These polymers can be engineered to facilitate the removal of contaminants from water sources, ensuring safe drinking water and promoting public health.
Several purification methods incorporate biocompatible polymers:
- Membrane Filtration: Membranes made from biocompatible materials can effectively filter out pathogens and impurities. Their inherent properties allow them to operate efficiently in various conditions.
- Adsorption Techniques: Polymers can be designed to absorb toxins or heavy metals from water, providing a low-cost solution for water treatment facilities.
Innovation in this field is crucial for developing cost-effective and efficient systems. The use of biocompatible materials in water treatment technologies not only provides environmental benefits but also enhances the recycling of materials, contributing to overall sustainability.
In summary, the role of biocompatible polymers in environmental applications is becoming increasingly significant. By providing alternatives to conventional materials and enhancing purification processes, these polymers pave the way for a more sustainable future.
Polymer Synthesis Methods
The synthesis methods of polymers are crucial in developing biocompatible materials. These methods lay the foundation for designing polymers with specific properties tailored for applications in medicine and environmental technology. Understanding the various processes involved in polymer synthesis enables researchers to better utilize biocompatible materials to address critical challenges in these fields.
Natural Polymer Extraction
Natural polymers, sourced from biological materials, play an essential role in various applications, particularly in biomedical fields. The extraction of natural polymers often involves procedures like solvent extraction, precipitation, and purification. This process is significant as it ensures the integrity and functionality of the polymer. A key advantage of natural polymer extraction is that the resulting materials can often interact harmoniously with biological tissues, minimizing the risk of adverse reactions. Additionally, natural polymers are typically biodegradable, which aligns with current trends towards sustainability in material science. However, challenges such as variability in source materials and extraction efficiency can affect the quality and consistency of natural polymers.
Synthetic Polymerization Techniques
Synthetic polymerization techniques are essential for producing tailored biocompatible materials. Two primary methods dominate this domain: addition polymerization and condensation polymerization.
Addition Polymerization
Addition polymerization is a method where monomers with unsaturated bonds link together to form polymers without the loss of any small molecules. A key characteristic of this process lies in its versatility; it allows for the production of a wide range of polymers with diverse properties. The approach is beneficial for this article because it facilitates the creation of high-performance materials ideal for medical applications like drug delivery systems and tissue engineering. One unique feature of addition polymerization is its ability to control molecular weights and the architecture of the polymer chains, providing significant advantages in customizing performance. Still, the method may have disadvantages, such as environmental concerns related to specific catalysts used in the process.
Condensation Polymerization
Condensation polymerization involves the reaction of monomers that react with the elimination of small molecules, typically water. This method is prominent in generating polymers with enhanced thermal stability and mechanical strength. A significant feature of condensation polymerization is its ability to create complex structures with various functional groups. This characteristic makes condensation polymers quite popular in biomedical applications. Additionally, the broad range of possible resulting materials means they can be fine-tuned to specific applications. However, the main disadvantage is that residual small molecules can become impurities, affecting the biocompatibility of the final product.
In summary, both natural and synthetic polymerization techniques provide paths to develop biocompatible polymers with applications in medicine and the environment. A thorough understanding of these methods allows for innovation and growth in the field, addressing future research and practical applications.
Regulatory Considerations
The landscape of biocompatible polymers is greatly shaped by regulatory considerations. These guidelines play a pivotal role in ensuring that the materials developed meet safety and efficacy standards for various applications. The regulatory framework helps mitigate risks associated with the use of these polymers in sensitive areas such as medicine and environmental applications.
Furthermore, regulations ensure that the right testing methods are employed to assess biocompatibility, toxicity, and long-term stability. This ensures that innovations in biopolymers can be safely transitioned into real-world applications without compromising human health or the ecosystem.
To effectively navigate this complex regulatory terrain, it is crucial for researchers and manufacturers to be well-acquainted with relevant guidelines set by authoritative bodies.
FDA Guidelines
The Food and Drug Administration (FDA) plays a central role in the regulation of biocompatible polymers used in medical applications. Their guidelines outline a comprehensive approach for evaluating the safety and effectiveness of medical devices that utilize these materials.
Key aspects of the FDA guidelines include:
- Pre-market Submission: Manufacturers must submit a pre-market approval or a 510(k) notification demonstrating that their products are safe and effective for human use.
- Biocompatibility Testing: Specific tests may be required to evaluate the interaction of polymers with biological systems, which can include cytotoxicity, sensitization, and irritation assessments.
- Post-market Surveillance: Ongoing monitoring of the biocompatible polymer products in the market is essential to track and manage any potential adverse effects.
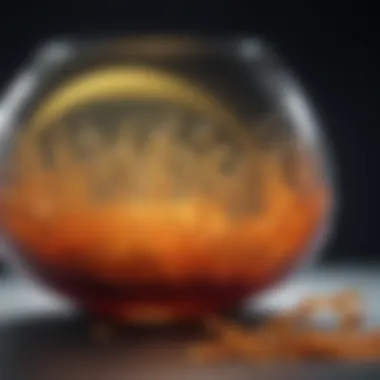
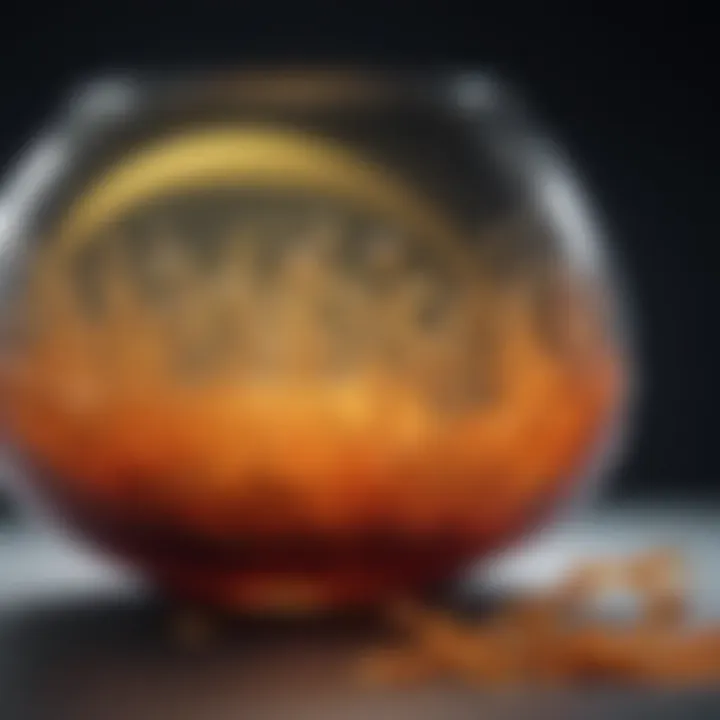
By adhering to FDA guidelines, researchers can ensure that their innovations not only advance science but also protect public health.
ISO Standards
The International Organization for Standardization (ISO) establishes global standards that help define the quality and reliability of biocompatible polymers. These standards facilitate trade and enhance consumer and regulatory confidence in the products.
Important ISO standards relevant to biocompatible polymers include:
- ISO 10993: This is a series of standards that outlines the biological evaluation of medical devices to ensure biocompatibility. It specifies the necessary tests for evaluating local and systemic effects of materials used in devices.
- ISO 13485: This standard addresses the quality management systems for organizations involved in the design, production, installation, and servicing of medical devices. Adhering to this standard assures that the products meet both customer and regulatory requirements without objectionable risk.
Implementing these standards not only ensures compliance but also enhances the marketability of biocompatible polymers by demonstrating a commitment to quality and safety.
Challenges in Biocompatible Polymer Research
The exploration of biocompatible polymers is crucial to advancing both medical and environmental fields. Despite significant progress, researchers face various challenges in ensuring the stability, cost-effectiveness, and practicality of these materials. Addressing these issues is essential for the successful implementation of biocompatible polymers in real-world applications. This section delves into two primary challenges: material stability and the cost of production.
Material Stability
Material stability is a fundamental concern when discussing biocompatible polymers. These materials must maintain their properties and functionality over time, especially in biological environments. The degradation process can be influenced by factors such as temperature, pH, and the presence of enzymes. If a polymer degrades too quickly, it may not provide the intended therapeutic effects. Conversely, if it remains stable for too long, it can lead to adverse reactions in the body.
To ensure longevity and effectiveness, researchers continuously work on modifying chemical structures. Techniques like cross-linking can enhance stability, but this may also change other properties of the polymer. Finding the right balance is crucial. Key considerations in improving material stability include:
- Composition: Selecting the appropriate monomers can influence the polymer's resistance to environmental factors.
- Processing conditions: The method used to synthesize the polymers affects their stability, necessitating careful selection of parameters.
- Post-treatment: Various treatments can further enhance stability post-synthesis, such as the addition of stabilizers.
In summary, achieving optimal material stability requires a comprehensive understanding of the polymer's behavior under diverse conditions. Successful solutions can dramatically enhance the reliability of biocompatible polymers in various applications.
Cost of Production
The cost of production is another significant challenge in biocompatible polymer research. Many innovative materials, while effective and promising, are prohibitively expensive to produce at scale. The financial burden not only limits research but also restricts commercialization efforts. Several factors contribute to high production costs:
- Raw materials: Sourcing high-quality monomers can be costly. Natural polymers, while desirable for their biocompatibility, may have variable availability and higher costs compared to synthetic alternatives.
- Synthesis methods: Advanced polymerization techniques can increase manufacturing complexity and, thus, cost. There’s a constant push to discover both economically viable and efficient synthesis approaches.
- Quality control: Ensuring that biocompatible polymers meet regulatory standards requires rigorous testing, adding to overall expenses.
To tackle the issue of production costs, it is necessary to invest in research aimed at streamlining processes and exploring alternative, less expensive materials. Collaboration between academic researchers and industry can foster innovation and lead to more cost-effective solutions, allowing biocompatible polymers to reach a wider market.
Research must continue to focus on making biocompatible polymers not only effective but also economically viable to ensure their long-term success in various applications.
Innovations and Future Trends
In the realm of biocompatible polymers, staying abreast of innovations and future trends is crucial. These advancements not only enhance existing applications but also pave the way for novel solutions in diverse fields such as medicine and environmental science. Exploring smart polymers and nanotechnology, one can observe how these innovations improve efficacy and functionality.
Smart Polymers
Smart polymers, also known as stimuli-responsive polymers, showcase significant potential in various applications. These materials can change their properties based on external stimuli, such as temperature, pH, or light. Such behavior can lead to controlled drug release systems, making treatments more efficient and targeted. For example, a polymer that responds to body temperature can release medication at the affected area, minimizing side effects and enhancing therapeutic effects.
The shift towards using smart polymers reflects an increasing demand for personalization in medical treatments. Healthcare providers can better tailor therapies. Additionally, industries are exploring smart polymers for applications outside of medicine. In packaging, the ability to respond to environmental changes can indicate product freshness or integrity, similar to how it’s done in biomaterials.
Nanotechnology in Polymers
The integration of nanotechnology with biocompatible polymers is transforming the landscape of material science. Nanoparticles can enhance properties such as strength, conductivity, and bioactivity. This incorporation allows for the development of safer, more efficient drugs and improved delivery systems. For instance, nanoparticles can help traverse biological barriers, ensuring medications reach their intended targets in the body efficiently.
Moreover, nanotechnology can result in improved degradation rates for biodegradable polymers. This advancement is pivotal in environmental applications, such as water purification systems. The use of nanostructured materials can significantly enhance filtration capabilities, responding to growing concerns over water scarcity and pollution.
End
The conclusion of this article highlights the critical role that biocompatible polymers play in both medical and environmental applications. This summary not only encapsulates the main points discussed but also reflects on the significance of these materials in improving health outcomes and addressing ecological challenges.
Summary of Key Points
Biocompatible polymers are essential in various applications that impact life significantly. They are categorized into natural and synthetic types, each offering unique properties and advantages. Some key points include:
- Definition of Biocompatibility: This term describes how well a material interacts with biological systems without causing adverse reactions.
- Diverse Applications: From drug delivery systems to tissue engineering, these polymers facilitate modern medical practices.
- Synthesis Methods: Natural extraction and synthetic polymerization techniques are crucial for developing effective biocompatible materials.
- Regulatory Standards: Compliance with FDA guidelines and ISO standards ensures the safety and effectiveness of these materials in medical use.
- Challenges and Innovations: Ongoing research addresses issues like material stability and production costs, which can potentially limit the application of biocompatible polymers.
Implications for Future Research
Future research in the field of biocompatible polymers holds substantial implications for advancing medical technology and environmental solutions. Areas to explore include:
- Smart Polymers: The development of responsive polymers that can adapt to environmental changes or stimuli.
- Nanotechnology Applications: The integration of nanotechnology could lead to enhanced performance and new functionalities in biocompatible polymers.
- Sustainability Concerns: Research aimed at improving the sustainability of polymer production and exploring biodegradable options is imperative for environmental health.
- Clinical Applications: Continued investigation is necessary to assess the long-term effects of biocompatible materials in human bodies and their role in regenerative medicine.
The exploration of these implications will not only advance existing knowledge but also foster innovation in the use of biocompatible polymers, ultimately reinforcing their value in contemporary science and medicine.